Mutation of the Fucose-Specific 1,3 N-Acetylglucosaminyltransferase
Total Page:16
File Type:pdf, Size:1020Kb
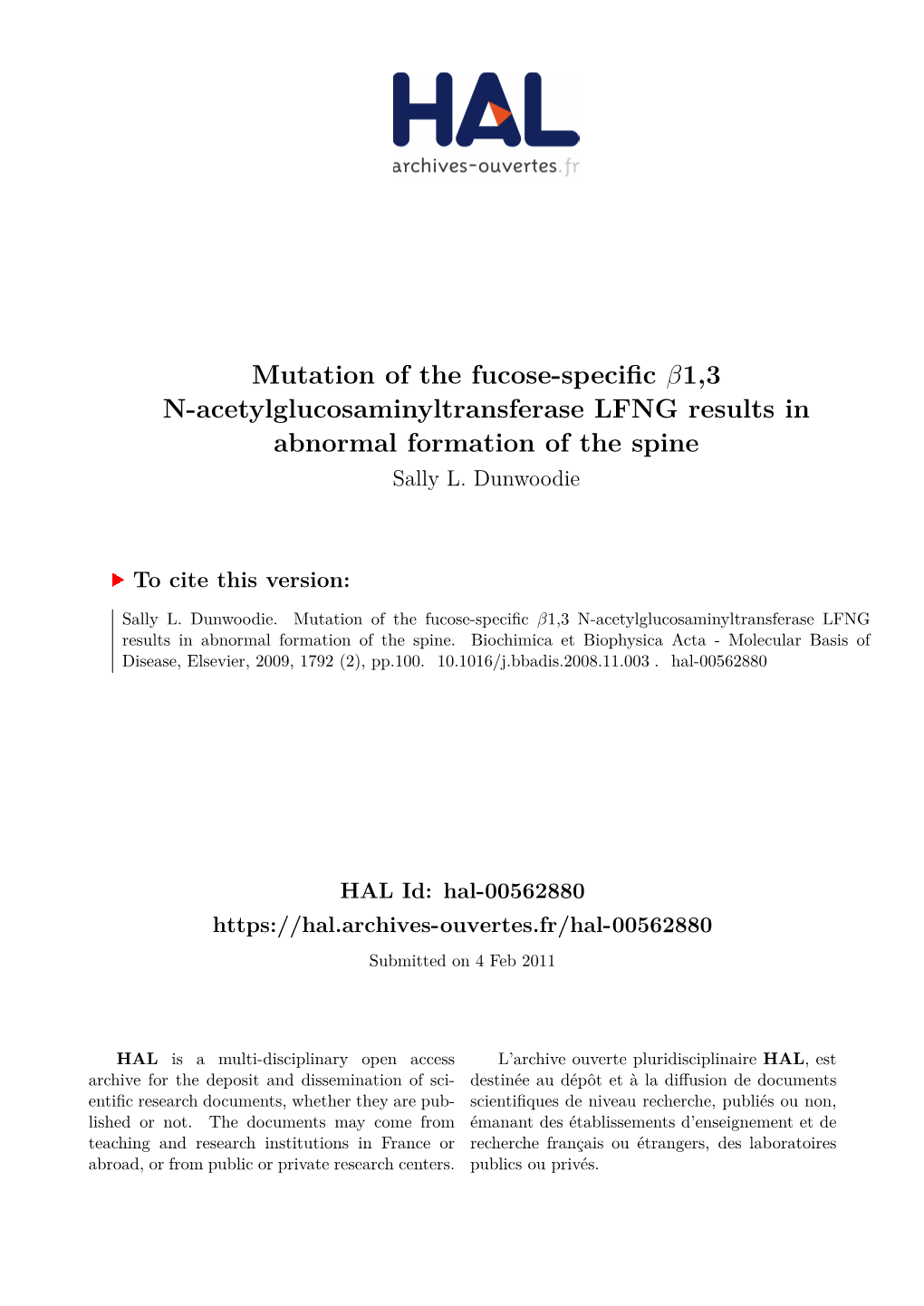
Load more
Recommended publications
-
Anti-Lunatic Fringe Antibody (ARG40136)
Product datasheet [email protected] ARG40136 Package: 100 μl anti-Lunatic Fringe antibody Store at: -20°C Summary Product Description Rabbit Polyclonal antibody recognizes Lunatic Fringe Tested Reactivity Hu Tested Application FACS, IHC-P, WB Host Rabbit Clonality Polyclonal Isotype IgG Target Name Lunatic Fringe Antigen Species Human Immunogen KLH-conjugated synthetic peptide corresponding to aa. 86-114 of Human Lunatic Fringe. Conjugation Un-conjugated Alternate Names O-fucosylpeptide 3-beta-N-acetylglucosaminyltransferase; EC 2.4.1.222; SCDO3; Beta-1,3-N- acetylglucosaminyltransferase lunatic fringe Application Instructions Application table Application Dilution FACS 1:10 - 1:50 IHC-P 1:50 - 1:100 WB 1:1000 Application Note * The dilutions indicate recommended starting dilutions and the optimal dilutions or concentrations should be determined by the scientist. Positive Control K562 Calculated Mw 42 kDa Properties Form Liquid Purification Purification with Protein A and immunogen peptide. Buffer PBS and 0.09% (W/V) Sodium azide. Preservative 0.09% (W/V) Sodium azide. Storage instruction For continuous use, store undiluted antibody at 2-8°C for up to a week. For long-term storage, aliquot and store at -20°C or below. Storage in frost free freezers is not recommended. Avoid repeated freeze/thaw cycles. Suggest spin the vial prior to opening. The antibody solution should be gently mixed before use. www.arigobio.com 1/3 Note For laboratory research only, not for drug, diagnostic or other use. Bioinformation Gene Symbol LFNG Gene Full Name LFNG O-fucosylpeptide 3-beta-N-acetylglucosaminyltransferase Background This gene is a member of the fringe gene family which also includes radical and manic fringe genes. -
Segmentation in Vertebrates: Clock and Gradient Finally Joined
Downloaded from genesdev.cshlp.org on September 24, 2021 - Published by Cold Spring Harbor Laboratory Press REVIEW Segmentation in vertebrates: clock and gradient finally joined Alexander Aulehla1 and Bernhard G. Herrmann2 Max-Planck-Institute for Molecular Genetics, Department of Developmental Genetics, 14195 Berlin, Germany The vertebral column is derived from somites formed by terior (A–P) axis. Somite formation takes place periodi- segmentation of presomitic mesoderm, a fundamental cally in a fixed anterior-to-posterior sequence. process of vertebrate embryogenesis. Models on the In the chick embryo, a new somite is formed approxi- mechanism controlling this process date back some mately every 90 min, whereas in the mouse embryo, the three to four decades. Access to understanding the mo- periodicity varies, dependent on the axial position (Tam lecular control of somitogenesis has been gained only 1981). Classical embryology experiments revealed that recently by the discovery of molecular oscillators (seg- periodicity and directionality of somite formation are mentation clock) and gradients of signaling molecules, controlled by an intrinsic program set off in the cells as as predicted by early models. The Notch signaling path- they are recruited into the psm. For instance, when the way is linked to the oscillator and plays a decisive role in psm is inverted rostro–caudally, somite formation in the inter- and intrasomitic boundary formation. An Fgf8 sig- inverted region proceeds from caudal to rostral, main- naling gradient is involved in somite size control. And taining the original direction (Christ et al. 1974). More- the (canonical) Wnt signaling pathway, driven by Wnt3a, over, neither the transversal bisection nor the isolation appears to integrate clock and gradient in a global of the psm from all surrounding tissues stops the seg- mechanism controlling the segmentation process. -
The Regulation of Lunatic Fringe During Somitogenesis
THE REGULATION OF LUNATIC FRINGE DURING SOMITOGENESIS DISSERTATION Presented in Partial Fulfillment of the Requirements for the Degree Doctor of Philosophy in the Graduate School of The Ohio State University By Emily T. Shifley ***** The Ohio State University 2009 Dissertation Committee: Approved by Professor Susan Cole, Advisor Professor Christine Beattie _________________________________ Professor Mark Seeger Advisor Graduate Program in Molecular Genetics Professor Michael Weinstein ABSTRACT Somitogenesis is the morphological hallmark of vertebrate segmentation. Somites bud from the presomitic mesoderm (PSM) in a sequential, periodic fashion and give rise to the rib cage, vertebrae, and dermis and muscles of the back. The regulation of somitogenesis is complex. In the posterior region of the PSM, a segmentation clock operates to organize cohorts of cells into presomites, while in the anterior region of the PSM the presomites are patterned into rostral and caudal compartments (R/C patterning). Both of these stages of somitogenesis are controlled, at least in part, by the Notch pathway and Lunatic fringe (Lfng), a glycosyltransferase that modifies the Notch receptor. To dissect the roles played by Lfng during somitogenesis, we created a novel allele that lacks cyclic Lfng expression within the segmentation clock, but that maintains expression during R/C somite patterning (Lfng∆FCE1). Lfng∆FCE1/∆FCE1 mice have severe defects in their anterior vertebrae and rib cages, but relatively normal sacral and tail vertebrae, unlike Lfng knockouts. Segmentation clock function is differentially affected by the ∆FCE1 deletion; during anterior somitogenesis the expression patterns of many clock genes are disrupted, while during posterior somitogenesis, certain clock components have recovered. R/C patterning occurs relatively normally in Lfng∆FCE1/∆FCE1 embryos, likely contributing to the partial phenotype rescue, and confirming that Lfng ii plays separate roles in the two regions of the PSM. -
Neural Development
31 October 2007 NEURAL DEVELOPMENT www.neuraldevelopment.com Analysis of Lrrn1 expression and its relationship to neuromeric boundaries during chick neural development Laura C Andreae et al. Neural Development 2007, 2:22 http://www.neuraldevelopment.com/content/2/1/22 Neural Development BioMed Central Research article Open Access Analysis of Lrrn1 expression and its relationship to neuromeric boundaries during chick neural development LauraCAndreae1,2, Daniela Peukert1, Andrew Lumsden1 and Jonathan D Gilthorpe*1 Address: 1MRC Centre for Developmental Neurobiology, King's College London, New Hunt's House, Guy's Campus, London, UK, SE1 1UL and 2Department of Neurophysiology, National Institute for Medical Research, The Ridgeway, Mill Hill, London, UK, NW7 1AA Email: Laura C Andreae - [email protected]; Daniela Peukert - [email protected]; Andrew Lumsden - [email protected]; Jonathan D Gilthorpe* - [email protected] * Corresponding author Published: 31 October 2007 Received: 26 March 2007 Accepted: 31 October 2007 Neural Development 2007, 2:22 doi:10.1186/1749-8104-2-22 This article is available from: http://www.neuraldevelopment.com/content/2/1/22 © 2007 Andreae et al.; licensee BioMed Central Ltd. This is an open access article distributed under the terms of the Creative Commons Attribution License (http://creativecommons.org/licenses/by/2.0), which permits unrestricted use, distribution, and reproduction in any medium, provided the original work is properly cited. Abstract Background: The Drosophila leucine-rich repeat proteins Tartan (TRN) and Capricious (CAPS) mediate cell affinity differences during compartition of the wing imaginal disc. This study aims to identify and characterize the expression of a chick orthologue of TRN/CAPS and examine its potential function in relation to compartment boundaries in the vertebrate central nervous system. -
Downloaded Per Proteome Cohort Via the Web- Site Links of Table 1, Also Providing Information on the Deposited Spectral Datasets
www.nature.com/scientificreports OPEN Assessment of a complete and classifed platelet proteome from genome‑wide transcripts of human platelets and megakaryocytes covering platelet functions Jingnan Huang1,2*, Frauke Swieringa1,2,9, Fiorella A. Solari2,9, Isabella Provenzale1, Luigi Grassi3, Ilaria De Simone1, Constance C. F. M. J. Baaten1,4, Rachel Cavill5, Albert Sickmann2,6,7,9, Mattia Frontini3,8,9 & Johan W. M. Heemskerk1,9* Novel platelet and megakaryocyte transcriptome analysis allows prediction of the full or theoretical proteome of a representative human platelet. Here, we integrated the established platelet proteomes from six cohorts of healthy subjects, encompassing 5.2 k proteins, with two novel genome‑wide transcriptomes (57.8 k mRNAs). For 14.8 k protein‑coding transcripts, we assigned the proteins to 21 UniProt‑based classes, based on their preferential intracellular localization and presumed function. This classifed transcriptome‑proteome profle of platelets revealed: (i) Absence of 37.2 k genome‑ wide transcripts. (ii) High quantitative similarity of platelet and megakaryocyte transcriptomes (R = 0.75) for 14.8 k protein‑coding genes, but not for 3.8 k RNA genes or 1.9 k pseudogenes (R = 0.43–0.54), suggesting redistribution of mRNAs upon platelet shedding from megakaryocytes. (iii) Copy numbers of 3.5 k proteins that were restricted in size by the corresponding transcript levels (iv) Near complete coverage of identifed proteins in the relevant transcriptome (log2fpkm > 0.20) except for plasma‑derived secretory proteins, pointing to adhesion and uptake of such proteins. (v) Underrepresentation in the identifed proteome of nuclear‑related, membrane and signaling proteins, as well proteins with low‑level transcripts. -
EXAMINING the POST-TRANSCRIPTIONAL REGULATION of LUNATIC FRINGE (Lfng) in the MOUSE SEGMENTATION CLOCK
EXAMINING THE POST-TRANSCRIPTIONAL REGULATION OF LUNATIC FRINGE (Lfng) IN THE MOUSE SEGMENTATION CLOCK DISSERTATION Presented in Partial Fulfillment of the Requirements for the Degree Doctor of Philosophy in the Graduate School of The Ohio State University By Kanu Wahi Graduate Program in Molecular Genetics The Ohio State University 2016 Dissertation Committee: Dr. Susan Cole, Advisor Dr. Keith Slotkin Dr. Robin Wharton Dr. Dawn Chandler Copyright by Kanu Wahi 2016 Abstract Somitogenesis is a developmental process in vertebrates involving periodic formation of somites that bud from an unsegmented region known as the pre-somitic mesoderm (PSM) and give rise to the axial skeleton and skeletal muscle in the developed organism. The process of somitogenesis is regulated by a "segmentation clock" that times somite formation and is evolutionarily conserved among vertebrates. Genes, such as Lunatic fringe (Lfng), are required for normal clock function in chickens, mice and humans and exhibit rapid cyclic expression in the PSM with a period that matches the rate of somite formation. To maintain rapid oscillations, especially in the posterior PSM, it is hypothesized that the Lfng transcript should be promptly degraded to ensure its clearance of from cells before the next round of oscillation begins. The 3’UTR of Lfng contains number of conserved sequences that could regulate Lfng expression at the post transcriptional level. In this study we explore the post- transcriptional regulation of the Lfng transcript by the Lfng 3’UTR in the context of the segmentation clock. The Lfng 3'UTR has binding sites for microRNAs (miRNAs), such as miR-125a and miR-200b that are known to affect transcript stability via the 3’UTR. -
Fine-Tuning of Notch Signaling Sets the Boundary of the Organ of Corti And
RESEARCH ARTICLE Fine-tuning of Notch signaling sets the boundary of the organ of Corti and establishes sensory cell fates Martin L Basch1†, Rogers M Brown II2, Hsin-I Jen2, Fatih Semerci2, Frederic Depreux3, Rene´ e K Edlund2, Hongyuan Zhang1, Christine R Norton4, Thomas Gridley4, Susan E Cole5, Angelika Doetzlhofer6, Mirjana Maletic-Savatic1,2,7,8, Neil Segil9, Andrew K Groves1,2,10* 1Department of Neuroscience, Baylor College of Medicine, Houston, United States; 2Program in Developmental Biology, Baylor College of Medicine, Houston, United States; 3Department of Cell Biology and Anatomy, Rosalind Franklin University of Medicine and Science, Chicago, United States; 4Maine Medical Center Research Institute, Scarborough, United States; 5Department of Molecular Genetics, The Ohio State University, Columbus, United States; 6Solomon H. Snyder Department of Neuroscience, Johns Hopkins University, School of Medicine, Baltimore, United States; 7Department of Pediatrics, Baylor College of Medicine, Houston, United States; 8Jan and Dan Duncan Neurological Research Institute at Texas Children’s Hospital, Houston, United States; 9Department of Stem Cell Biology and Regenerative Medicine, Keck School of Medicine, University of Southern California, Los Angeles, United States; 10Department of Molecular and Human Genetics, Baylor *For correspondence: akgroves@ College of Medicine, Houston, United States bcm.edu Present address: †Department of Otolaryngology Head and Neck Surgery, University Abstract The signals that induce the organ of Corti and define its boundaries in the cochlea are Hospitals, Case Medical Center, poorly understood. We show that two Notch modifiers, Lfng and Mfng, are transiently expressed Case Western Reserve University, precisely at the neural boundary of the organ of Corti. Cre-Lox fate mapping shows this region Cleveland, United States gives rise to inner hair cells and their associated inner phalangeal cells. -
Datasheet: AHP2266 Product Details
Datasheet: AHP2266 Description: GOAT ANTI HUMAN MANIC FRINGE Specificity: MANIC FRINGE Other names: MFNG Format: Purified Product Type: Polyclonal Antibody Isotype: Polyclonal IgG Quantity: 0.1 mg Product Details Applications This product has been reported to work in the following applications. This information is derived from testing within our laboratories, peer-reviewed publications or personal communications from the originators. Please refer to references indicated for further information. For general protocol recommendations, please visit www.bio-rad-antibodies.com/protocols. Yes No Not Determined Suggested Dilution Flow Cytometry Immunohistology - Frozen Immunohistology - Paraffin (1) 2.0 - 4.0ug/ml ELISA 1/8000 Immunoprecipitation Western Blotting Where this product has not been tested for use in a particular technique this does not necessarily exclude its use in such procedures. Suggested working dilutions are given as a guide only. It is recommended that the user titrates the product for use in their own system using appropriate negative/positive controls. (1)This product requires antigen retrieval using heat treatment prior to staining of paraffin sections.Tris/EDTA buffer pH 9.0 is recommended for this purpose. Target Species Human Species Cross Based on sequence similarity, is expected to react with:Bovine Reactivity N.B. Antibody reactivity and working conditions may vary between species. Product Form Purified IgG - liquid Antiserum Preparation Antiserum to human MFNG was raised by repeated immunisation of goats with highly -
Master Regulators, Regulatory Networks, and Pathways of Glioblastoma Subtypes Serdar Bozdag Marquette University, [email protected]
Marquette University e-Publications@Marquette Mathematics, Statistics and Computer Science, MSCS Faculty Research and Publications Department of 1-1-2014 Master Regulators, Regulatory Networks, and Pathways of Glioblastoma Subtypes Serdar Bozdag Marquette University, [email protected] Aiguo Li National Institutes of Health Mehmet Baysan National Institutes of Health Howard A. Fine National Institutes of Health Published version. Cancer Informatics, Vol. 13, S. 3 (2014): 33-44. DOI. This is an open-access article distributed under the terms of the Creative Commons CC-BY-NC 3.0 License. Open Access: Full open access to Cancer this and thousands of other papers at http://www.la-press.com. Informatics Supplementary Issue: Computational Advances in Cancer Informatics (B) Master Regulators, Regulatory Networks, and Pathways of Glioblastoma Subtypes Serdar Bozdag1,2, Aiguo Li1, Mehmet Baysan1,3 and Howard A. Fine1,3 1Neuro-Oncology Branch, National Cancer Institute, National Institute of Neurological Disorders and Stroke, National Institutes of Health, Bethesda, Maryland, USA. 2Department of Mathematics, Statistics, and Computer Science, Marquette University, Milwaukee, Wisconsin, USA. 3New York University Cancer Institute, New York University Langone Medical Center, New York, New York, USA. ABSTR ACT: Glioblastoma multiforme (GBM) is the most common malignant brain tumor. GBM samples are classified into subtypes based on their tran- scriptomic and epigenetic profiles. Despite numerous studies to better characterize GBM biology, a comprehensive study to identify GBM subtype- specific master regulators, gene regulatory networks, and pathways is missing. Here, we used FastMEDUSA to compute master regulators and gene regulatory networks for each GBM subtype. We also ran Gene Set Enrichment Analysis and Ingenuity Pathway Analysis on GBM expression dataset from The Cancer Genome Atlas Project to compute GBM- and GBM subtype-specific pathways. -
Table S1. 103 Ferroptosis-Related Genes Retrieved from the Genecards
Table S1. 103 ferroptosis-related genes retrieved from the GeneCards. Gene Symbol Description Category GPX4 Glutathione Peroxidase 4 Protein Coding AIFM2 Apoptosis Inducing Factor Mitochondria Associated 2 Protein Coding TP53 Tumor Protein P53 Protein Coding ACSL4 Acyl-CoA Synthetase Long Chain Family Member 4 Protein Coding SLC7A11 Solute Carrier Family 7 Member 11 Protein Coding VDAC2 Voltage Dependent Anion Channel 2 Protein Coding VDAC3 Voltage Dependent Anion Channel 3 Protein Coding ATG5 Autophagy Related 5 Protein Coding ATG7 Autophagy Related 7 Protein Coding NCOA4 Nuclear Receptor Coactivator 4 Protein Coding HMOX1 Heme Oxygenase 1 Protein Coding SLC3A2 Solute Carrier Family 3 Member 2 Protein Coding ALOX15 Arachidonate 15-Lipoxygenase Protein Coding BECN1 Beclin 1 Protein Coding PRKAA1 Protein Kinase AMP-Activated Catalytic Subunit Alpha 1 Protein Coding SAT1 Spermidine/Spermine N1-Acetyltransferase 1 Protein Coding NF2 Neurofibromin 2 Protein Coding YAP1 Yes1 Associated Transcriptional Regulator Protein Coding FTH1 Ferritin Heavy Chain 1 Protein Coding TF Transferrin Protein Coding TFRC Transferrin Receptor Protein Coding FTL Ferritin Light Chain Protein Coding CYBB Cytochrome B-245 Beta Chain Protein Coding GSS Glutathione Synthetase Protein Coding CP Ceruloplasmin Protein Coding PRNP Prion Protein Protein Coding SLC11A2 Solute Carrier Family 11 Member 2 Protein Coding SLC40A1 Solute Carrier Family 40 Member 1 Protein Coding STEAP3 STEAP3 Metalloreductase Protein Coding ACSL1 Acyl-CoA Synthetase Long Chain Family Member 1 Protein -
Fringe Proteins Modulate Notch-Ligand Cis and Trans
RESEARCH ARTICLE elifesciences.org Fringe proteins modulate Notch-ligand cis and trans interactions to specify signaling states Lauren LeBon1,2, Tom V Lee3, David Sprinzak4, Hamed Jafar-Nejad3, Michael B Elowitz1,2* 1Howard Hughes Medical Institute, California Institute of Technology, Pasadena, United States; 2Division of Biology and Biological Engineering, California Institute of Technology, Pasadena, United States; 3Department of Molecular and Human Genetics, Baylor College of Medicine, Houston, United States; 4Department of Biochemistry and Molecular Biology, Tel Aviv University, Tel Aviv, Israel Abstract The Notch signaling pathway consists of multiple types of receptors and ligands, whose interactions can be tuned by Fringe glycosyltransferases. A major challenge is to determine how these components control the specificity and directionality of Notch signaling in developmental contexts. Here, we analyzed same-cell (cis) Notch-ligand interactions for Notch1, Dll1, and Jag1, and their dependence on Fringe protein expression in mammalian cells. We found that Dll1 and Jag1 can cis-inhibit Notch1, and Fringe proteins modulate these interactions in a way that parallels their effects on trans interactions. Fringe similarly modulated Notch-ligand cis interactions during Drosophila development. Based on these and previously identified interactions, we show how the design of the Notch signaling pathway leads to a restricted repertoire of signaling states that promote heterotypic signaling between distinct cell types, providing insight into the design principles of the Notch signaling system, and the specific developmental process ofDrosophila dorsal-ventral boundary formation. DOI: 10.7554/eLife.02950.001 *For correspondence: [email protected] Competing interests: The authors declare that no Introduction competing interests exist. The Notch signaling pathway mediates communication between adjacent cells (Artavanis-Tsakonas et al., 1999). -
S41467-018-05074-Y.Pdf
ARTICLE DOI: 10.1038/s41467-018-05074-y OPEN Identification of susceptibility pathways for the role of chromosome 15q25.1 in modifying lung cancer risk Xuemei Ji et al.# Genome-wide association studies (GWAS) identified the chromosome 15q25.1 locus as a leading susceptibility region for lung cancer. However, the pathogenic pathways, through which sus- 1234567890():,; ceptibility SNPs within chromosome 15q25.1 affects lung cancer risk, have not been explored. We analyzed three cohorts with GWAS data consisting 42,901 individuals and lung expression quantitative trait loci (eQTL) data on 409 individuals to identify and validate the underlying pathways and to investigate the combined effect of genes from the identified susceptibility pathways. The KEGG neuroactive ligand receptor interaction pathway, two Reactome pathways, and 22 Gene Ontology terms were identified and replicated to be significantly associated with lung cancer risk, with P values less than 0.05 and FDR less than 0.1. Functional annotation of eQTL analysis results showed that the neuroactive ligand receptor interaction pathway and gated channel activity were involved in lung cancer risk. These pathways provide important insights for the etiology of lung cancer. Correspondence and requests for materials should be addressed to C.I.A. (email: [email protected]). #A full list of authors and their affliations appears at the end of the paper. NATURE COMMUNICATIONS | (2018) 9:3221 | DOI: 10.1038/s41467-018-05074-y | www.nature.com/naturecommunications 1 ARTICLE NATURE COMMUNICATIONS | DOI: 10.1038/s41467-018-05074-y ung cancer, accounting for 13% of all cancer cases and 23% Results Lof all cancer-related deaths worldwide, is a leading cause of The study design is presented in Fig.