The Gravity Field of the Papuan Fold Belt and Its Geological Implications
Total Page:16
File Type:pdf, Size:1020Kb
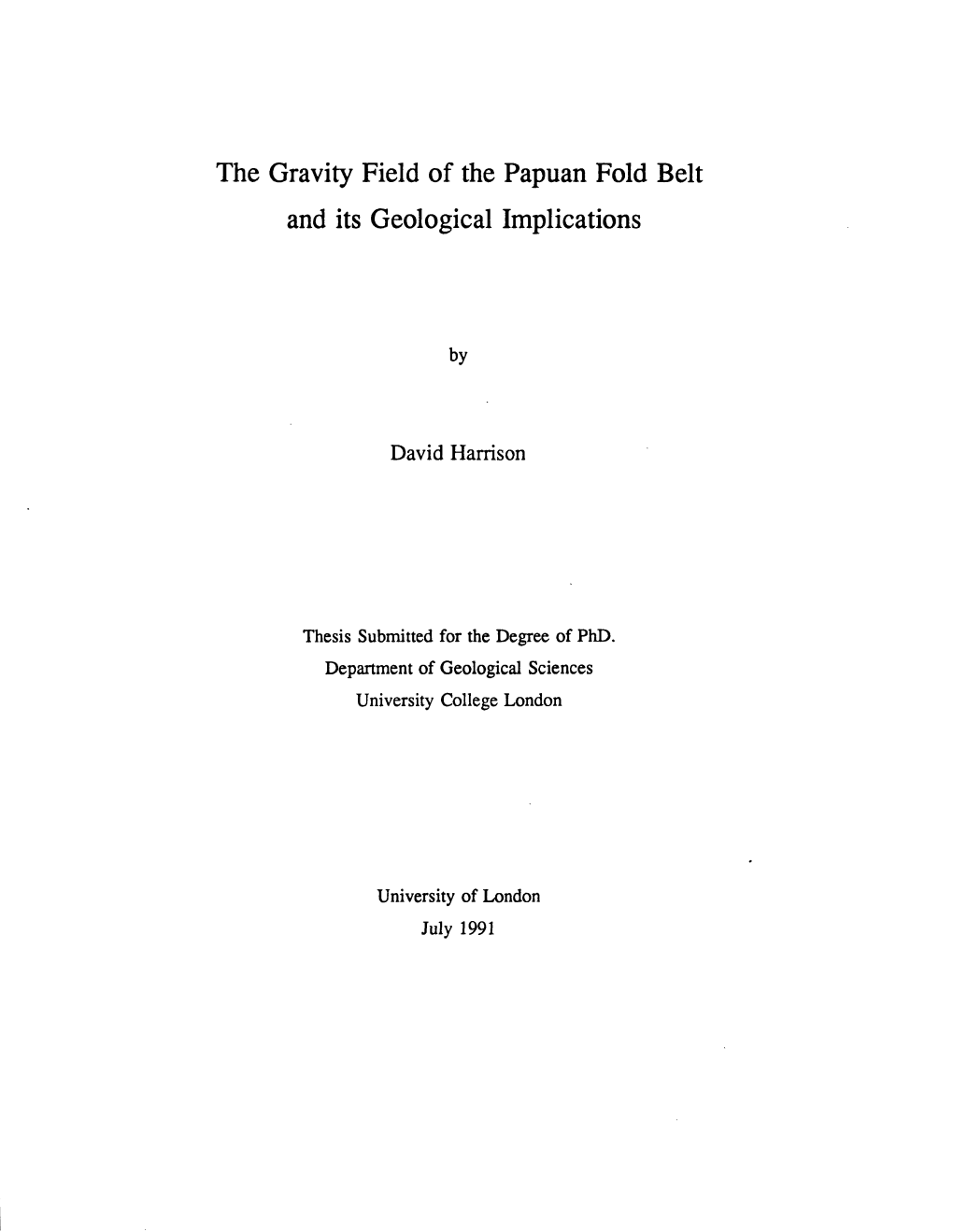
Load more
Recommended publications
-
Half Year Report (Due 31 October Each Year)
Darwin Initiative for the Survival of Species Half Year Report (due 31 October each year) Project Ref. No. 13-012 Project Title Integrated River Basin Management in the Sepik River Country(ies) Papua New Guinea UK WWF-UK Organisation Collaborator(s) PNG Department of Environment and Conservation, Ambunti Local Level Government, Ambunti District Local Environment Foundation (ADLEF), and project area communities Report date 1 April - 30 September 2004 Report No. 1 (HYR 1/2/3/4) Project website http://www.wwfpacific.org.fj/wetsepik.htm 1. Outline progress over the last 6 months (April – September) against the agreed baseline timetable for the project (if your project has started less than 6 months ago, please report on the period since start up). Progress towards project milestones: i) Confirm partnership agreements (Q1): The confirmation of partnership agreements between various partners and stakeholders in the national and local level has shown positive affirmation of better working relations. WWF PNG established and maintained the collaboration between the PNG Department of Environment and Conservation and Ambunti District Local Environment Foundation (ADLEF). WWF also strengthened the partnership network with other NGO’s and government institutions such as the PNG Department of Agriculture and Livestock (DAL), provincial government, local level government, and most importantly the local communities. This has enabled the better communication and facilitation of community planning and awareness mechanisms for the indigenous communities. ii) Consultants and staff hired (Q1-2): A position description for the WWF PNG Freshwater Programme Manager was formulated A copy is attached (Attachment A). The position vacancy announcement for a Freshwater Programme Manager was advertised in the major PNG newspaper on 9 June. -
GPS Results from the Woodlark Rift, Papua New Guinea, Geochem
PUBLICATIONS Geochemistry, Geophysics, Geosystems RESEARCH ARTICLE Continental breakup and UHP rock exhumation in action: GPS 10.1002/2014GC005458 results from the Woodlark Rift, Papua New Guinea Special Section: Laura M. Wallace1, Susan Ellis2, Tim Little3, Paul Tregoning4, Neville Palmer2, Robert Rosa5, Lithospheric Evolution of Richard Stanaway6, John Oa7, Edwin Nidkombu7, and John Kwazi7 Cenozoic UHP Terranes: From Convergence to Extension 1Institute for Geophysics, University of Texas, Austin, Texas, USA, 2GNS Science, Lower Hutt, New Zealand, 3School of Geography, Environment, and Earth Sciences, Victoria University of Wellington, Wellington, New Zealand, 4Research 5 Key Points: School for the Earth Sciences, Australian National University, Canberra, ACT, Australia, Surveying Department, University 6 7 GPS reveals crustal deformation and of Technology, Lae, Papua New Guinea, Quickclose Pty. Ltd., Carlton, Victoria, Australia, PNG National Mapping Bureau, microplate kinematics in the Port Moresby, Papua New Guinea Woodlark Basin, SE Papua New Guinea Exhumation of UHP rocks in We show results from a network of campaign Global Positioning System (GPS) sites in the Wood- southeastern PNG is associated with Abstract active crustal extension lark Rift, southeastern Papua New Guinea, in a transition from seafloor spreading to continental rifting. GPS Our results demonstrate that low- velocities indicate anticlockwise rotation (at 2–2.7/Myr, relative to Australia) of crustal blocks north of the rift, angle normal faults can slip at rates producing 10–15 mm/yr of extension in the continental rift, increasing to 20–40 mm/yr of seafloor spreading of several mm/yr or more at the Woodlark Spreading Center. Extension in the continental rift is distributed among multiple structures. -
Notes on the Gulf Province Languages Overview
Notes on the Gulf Province languages Karl Franklin (Data Collected 1968-1973; this report collated 2011) Information compiled here is from notes that I collected between 1968 and 1973. Following the completion of my Ph.D. degree at the Australian National University in 1969, I was awarded a post-doctoral fellowship in 1970 to conduct a linguistic survey of the Gulf Province. In preparation for the survey I wrote a paper that was published as: Franklin, Karl J. 1968. Languages of the Gulf District: A Preview. Pacific Linguistics, Series A, 16.19-44. As a result of the linguistic survey in1970, I edited a book with ten chapters, written by eight different scholars (Franklin, Lloyd, MacDonald, Shaw, Wurm, Brown, Voorhoeve and Dutton). From this data I proposed a classification scheme for 33 languages. For specific details see: Franklin, Karl J. 1973 (ed.) The linguistic situation in the Gulf District and adjacent areas, Papua New Guinea. Pacific Linguistics, Series C, 26, x + 597 pp. Overview There are three sections in this paper. The first is a table that briefly outlines information on languages, dialects and villages of the Gulf Province. (Note that I cannot verify the spelling of each village/language due to differences between various sources.) The second section of the paper is an annotated bibliography and the third is an Appendix with notes from Annual Reports of the Territory of Papua. Source Notes Author/Language Woodward Annual pp. 19-22 by Woodward notes that: Report (AR) Four men of Pepeha were murdered by Kibeni; there is 1919-20:19- now friendly relations between Kirewa and Namau; 22 information on patrols to Ututi, Sirebi, and Kumukumu village on a whaleboat. -
The Plate Tectonics of Cenozoic SE Asia and the Distribution of Land and Sea
Cenozoic plate tectonics of SE Asia 99 The plate tectonics of Cenozoic SE Asia and the distribution of land and sea Robert Hall SE Asia Research Group, Department of Geology, Royal Holloway University of London, Egham, Surrey TW20 0EX, UK Email: robert*hall@gl*rhbnc*ac*uk Key words: SE Asia, SW Pacific, plate tectonics, Cenozoic Abstract Introduction A plate tectonic model for the development of SE Asia and For the geologist, SE Asia is one of the most the SW Pacific during the Cenozoic is based on palaeomag- intriguing areas of the Earth$ The mountains of netic data, spreading histories of marginal basins deduced the Alpine-Himalayan belt turn southwards into from ocean floor magnetic anomalies, and interpretation of geological data from the region There are three important Indochina and terminate in a region of continen- periods in regional development: at about 45 Ma, 25 Ma and tal archipelagos, island arcs and small ocean ba- 5 Ma At these times plate boundaries and motions changed, sins$ To the south, west and east the region is probably as a result of major collision events surrounded by island arcs where lithosphere of In the Eocene the collision of India with Asia caused an the Indian and Pacific oceans is being influx of Gondwana plants and animals into Asia Mountain building resulting from the collision led to major changes in subducted at high rates, accompanied by in- habitats, climate, and drainage systems, and promoted dis- tense seismicity and spectacular volcanic activ- persal from Gondwana via India into SE Asia as well -
GSA Bulletin: Tectonic Controls on Facies Transitions in an Oblique
Tectonic controls on facies transitions in an oblique collision: The western Solomon Sea, Papua New Guinea Joseph Galewsky Earth Science Department and Institute of Tectonics, University of California, Santa Cruz, Eli A. Silver } California 95064 ABSTRACT 1986). The tectonic history of many ancient TECTONIC SETTING mountain belts has been unraveled by careful The western Solomon Sea is the site of a clos- analysis of foreland basin deposits. For example, The modern Bismarck volcanic arc formed ing ocean basin and an incipient arc-continent analysis of the flysch sequences in the Alpine when subduction of the Solomon Sea plate be- collision between the Bismarck arc and the front ranges has provided a wealth of information neath the South Bismarck plate initiated, proba- Australian continental margin in Papua New about the paleogeography and geodynamic his- bly during late Miocene time (Musgrave, 1990). Guinea. Migrated seismic reflection profiles tory of the Alps (Caron et al., 1989). The Bismarck forearc contains the relict Finis- and HAWAII MR1 sidescan sonar data indi- Some observations suggest that foreland terre arc, a PaleogeneÐearliest Neogene volcanic cate that sedimentation within the Solomon basins eventually reach a steady state in which arc that was part of the larger Outer Melanesian Sea basin is controlled by topographic gradi- the accommodation space in the basin remains Arc. The Outer Melanesian Arc was built above ents generated by flexure of the Solomon Sea relatively constant despite continued overthrust- the West Melanesian Trench in response to plate. Turbidites delivered to the basin by the ing of the orogen (Covey, 1986), but the role of Pacific plate subduction beneath the Australian submarine Markham Canyon extend farther inherited basement topography on the strati- plate (Robinson, 1974). -
Omati River Right-Of-Way Communal Resource Plan
Esso Highlands Limited Papua New Guinea LNG Project Omati River Right-of-Way Communal Resource Plan PGHU-EH-SPZZZ-700002 Discipline Rev # Rev Date Description Prep By Endorsed Approved Checked 0 07 May 2012 Issued for Use See Next Page for Signatures “Unclassified” Information contained in this document is subject to use and disclosure restrictions under contract Unclassified PAPUA NEW GUINEA Omati River Right-of-Way, Communal Resource Plan LNG Project Page ii of 58 CONTENTS EXECUTIVE SUMMARY ........................................................................................................... 6 1.0 INTRODUCTION ....................................................................................................... 10 1.1 Project Area and Omati River Right-of-Way Villages ............................................. 10 1.2 Resettlement Goal ................................................................................................... 10 1.3 Sources of Information and Compliance Protocols ................................................ 11 2.0 PROJECT DESCRIPTION ........................................................................................ 12 2.1 Introduction .............................................................................................................. 12 2.2 Schedule .................................................................................................................. 12 2.3 Offshore Pipeline Construction ............................................................................... 12 -
Seismotectonic Model and Probabilistic Seismic Hazard Assessment for Papua New Guinea
Bulletin of Earthquake Engineering (2020) 18:6571–6605 https://doi.org/10.1007/s10518-020-00966-1 ORIGINAL RESEARCH Seismotectonic model and probabilistic seismic hazard assessment for Papua New Guinea Hadi Ghasemi1 · Phil Cummins1,2 · Graeme Weatherill3 · Chris McKee4 · Martyn Hazelwood1 · Trevor Allen1 Received: 18 March 2020 / Accepted: 24 September 2020 / Published online: 9 October 2020 © The Author(s) 2020 Abstract Papua New Guinea (PNG) lies in a belt of intense tectonic activity that experiences high levels of seismicity. Although this seismicity poses signifcant risks to society, the Building Code of PNG and its underpinning seismic loading requirements have not been revised since 1982. This study aims to partially address this gap by updating the seismic zoning map on which the earthquake loading component of the building code is based. We performed a new probabilistic seismic hazard assessment for PNG using the OpenQuake software developed by the Global Earthquake Model Foundation (Pagani et al. in Seism Res Lett 85(3):692–702, 2014). Among other enhancements, for the frst time together with background sources, individual fault sources are implemented to represent active major and microplate boundaries in the region to better constrain the earthquake-rate and seismic-source models. The seismic-source model also models intraslab, Wadati–Beniof zone seismicity in a more realistic way using a continuous slab volume to constrain the fnite ruptures of such events. The results suggest a high level of hazard in the coastal areas of the Huon Peninsula and the New Britain–Bougainville region, and a relatively low level of hazard in the southwestern part of mainland PNG. -
Final Frontier: Newly Discovered Species of New Guinea
REPORT 2011 Conservation Climate Change Sustainability Final Frontier: Newly discovered species of New Guinea (1998 - 2008) WWF Western Melanesia Programme Office Author: Christian Thompson (the green room) www.greenroomenvironmental.com, with contributions from Neil Stronach, Eric Verheij, Ted Mamu (WWF Western Melanesia), Susanne Schmitt and Mark Wright (WWF-UK), Design: Torva Thompson (the green room) Front cover photo: Varanus macraei © Lutz Obelgonner. This page: The low water in a river exposes the dry basin, at the end of the dry season in East Sepik province, Papua New Guinea. © Text 2011 WWF WWF is one of the world’s largest and most experienced independent conservation organisations, with over 5 million supporters and a global Network active in more than 100 countries. WWF’s mission is to stop the degradation of the planet’s natural environment and to build a future in which humans live in harmony with nature, by conserving the world’s biological diversity, ensuring that the use of renewable natural resources is sustainable, and promoting the reduction of pollution and wasteful consumption. © Brent Stirton / Getty images / WWF-UK © Brent Stirton / Getty Images / WWF-UK Closed-canopy rainforest in New Guinea. New Guinea is home to one of the world’s last unspoilt rainforests. This report FOREWORD: shows, it’s a place where remarkable new species are still being discovered today. As well as wildlife, New Guinea’s forests support the livelihoods of several hundred A VITAL YEAR indigenous cultures, and are vital to the country’s development. But they’re under FOR FORESTS threat. This year has been designated the International Year of Forests, and WWF is redoubling its efforts to protect forests for generations to come – in New Guinea, and all over the world. -
Volcano 101102 Aleutian Intra-Oceani
Index Page numbers in italic, refer to figures and those in bold refer to entries in tables. 34~ volcano 101,102 Manus Basin axial depths 32 Aleutian intra-oceanic subduction system bathymetry 32 characteristics 4 geochemical characteristics 33-34 location 3 geophysical characteristics 32-33 Amami Plateau 165 lava geochemistry 23, 25 Andaman Sea 208 opening rate 32 andesite 61 tectonic setting 30-32, 31 calc-alkalic andesite 61-63, 62 Mariana Trough compared to continental crust 68-69, 68 axial depth profile 36 magma type spatial variations 63, 66 bathymetry 36 magma mixing 63-67 geochemical characteristics 38-39 major and trace element characteristics 65 geophysical characteristics 37-38 Aoga Shima 189,190 lava geochemistry 24, 25 Aoso volcano 223, 226 tectonic setting 34-38, 35 Arafura Shelf 208 model development 39-42, 40 arc accretion in Taiwan and Ireland 83-85 study methods 20-21 arc magmatism, general characteristics 56-57 back-arc spreading 6 geochemical modelling 59 Banda Sea 208 incompatible element chemistry 58-61 Banggai Islands 209 volcano distribution 57-58, 57 Batanta 209 arc-continent collision model 81, 94-95 Bellingshausen Island 286, 287, 287 active continental margins 81-82 geochemical variations and volcano histories arc accretion in Taiwan and Ireland 83-85 294-295 arc crustal composition 82 major and trace element composition 290-292 birth of active continental margins 82-83 new isotope analyses 293 comparison of Mayo-Connemara with Taiwan Benham Plateau 165 collisional orogenies 93 Bird's Head 209 continuous arc -
Post 8Ma Reconstruction of Papua New Guinea and Solomon Islands
ÔØ ÅÒÙ×Ö ÔØ Post 8 Ma reconstruction of Papua New Guinea and Solomon Islands: Microplate tectonics in a convergent plate boundary setting Robert J. Holm, Gideon Rosenbaum, Simon W. Richards PII: S0012-8252(16)30050-2 DOI: doi: 10.1016/j.earscirev.2016.03.005 Reference: EARTH 2238 To appear in: Earth Science Reviews Received date: 14 October 2015 Revised date: 14 January 2016 Accepted date: 11 March 2016 Please cite this article as: Holm, Robert J., Rosenbaum, Gideon, Richards, Simon W., Post 8 Ma reconstruction of Papua New Guinea and Solomon Islands: Microplate tectonics in a convergent plate boundary setting, Earth Science Reviews (2016), doi: 10.1016/j.earscirev.2016.03.005 This is a PDF file of an unedited manuscript that has been accepted for publication. As a service to our customers we are providing this early version of the manuscript. The manuscript will undergo copyediting, typesetting, and review of the resulting proof before it is published in its final form. Please note that during the production process errors may be discovered which could affect the content, and all legal disclaimers that apply to the journal pertain. ACCEPTED MANUSCRIPT Post 8 Ma reconstruction of Papua New Guinea and Solomon Islands: Microplate tectonics in a convergent plate boundary setting Robert J. Holm 1, 2 , Gideon Rosenbaum 3, Simon W. Richards 1, 2 1Department of Earth and Oceans, College of Science, Technology & Engineering, James Cook University, Townsville, Queensland 4811, Australia 2Economic Geology Research Centre (EGRU), College of Science, Technology & Engineering, James Cook University, Townsville, Queensland 4811, Australia 3School of Earth Sciences, The University of Queensland, Brisbane, Queensland 4072, Australia corresponding author: [email protected] ABSTRACT Papua New Guinea and the Solomon Islands are located in a complex tectonic setting between the convergingACCEPTED Ontong Java Plateau MANUSCRIPT on the Pacific plate and the Australian continent. -
World-Heritage-Sites-Png
WORLD HERITAGE TENTATIVE LISTED SITES IN PAPUA NEW GUINEA REPORT ON A REVIEW OF THE SITES By Peter Hitchcock and Jennifer Gabriel January 2015 Photo Credit: Rodrick Vana, Oro Province REVIEW OF TENTATIVE WORLD HERITAGE SITES IN PAPUA NEW GUINEA Principal Authors Peter Hitchcock AM OCConsulting (Environment and Heritage) Cairns, Queensland Australia Contacts: P.O. Box 1133 Smithfield (Cairns) 4878 Tel: +61 (0)7 40381118 Mob: 0419 795 841 Email: [email protected] Jennifer Gabriel, B.Soc. Sc. (Hons. 1) PhD Scholar (Anthropology), Research Fellow - The Cairns Institute James Cook University Australia Assisted by Dr Matthew Leavesley FSA Adjunct Lecturer in Archaeology James Cook University Lecturer in Archaeology University of Papua New Guinea Dedication This report is dedicated to the memory of the late Mr. Vagi Renagi Genorupa, Manager, National World Heritage Secretariat, PNG Department of Environment and Conservation (d . 2nd December, 2014). 2 REVIEW OF TENTATIVE WORLD HERITAGE SITES IN PAPUA NEW GUINEA Background The Government of Papua New Guinea advised its acceptance of the World Heritage Convention on Monday, July 28, 1997. In advising it’s acceptance of the Convention, the Government of PNG joined other signatories in committing to, amongst other things, as far as possible to: 1. “adopt a general policy that aims to give the cultural and natural heritage a function in the life of the community and to integrate the protection of that heritage into comprehensive planning programs’; 2. undertake 'appropriate legal, scientific, technical, administrative and financial measures necessary for the identification, protection, conservation, presentation and rehabilitation of this heritage'; 3. refrain from 'any deliberate measures which might damage, directly or indirectly, the cultural and natural heritage' of other Parties to the Convention, and to help other Parties in the identification and protection of their properties.” UNESCO In accordance with Article 11 (1) of the Convention, in 2006 PNG formally nominated seven identified areas for Tentative Listing. -
Carettochelys Insculpta Ramsay 1886 – Pig-Nosed Turtle, Fly River Turtle
Conservation Biology of Freshwater Turtles and Tortoises: A Compilation ProjectCarettochelyidae of the IUCN/SSC — Tortoise Carettochelys and Freshwater insculpta Turtle Specialist Group 009.1 A.G.J. Rhodin, P.C.H. Pritchard, P.P. van Dijk, R.A. Saumure, K.A. Buhlmann, and J.B. Iverson, Eds. Chelonian Research Monographs (ISSN 1088-7105) No. 5, doi:10.3854/crm.5.009.insculpta.v1.2008 © 2008 by Chelonian Research Foundation • Published 13 June 2008 Carettochelys insculpta Ramsay 1886 – Pig-Nosed Turtle, Fly River Turtle ARTHUR GEOR G ES 1, J. SE A N DOO D Y 1, CA RL A EISEMBER G 1, ERIK A A. AL ac S 1, A N D MA RK ROSE 2 1Institute for Applied Ecology, University of Canberra, ACT 2601, Australia [[email protected]; [email protected]; [email protected]; [email protected]]; 2Fauna and Flora International, Jupiter House, 4th Floor, Station Road, Cambridge, CB1 2JD United Kingdom [[email protected]] SUMM A RY . – Carettochelys insculpta, the pig-nosed turtle (Family Carettochelyidae), is the sole surviving member of a family of turtles that was widely distributed during the Tertiary. It is re- stricted to the southern rivers of New Guinea and the rivers of the Northern Territory in Australia. Carettochelys is therefore a distinctive geographic and taxonomic relict and, although locally abun- dant, it is rare in the sense of being geographically restricted. Moreover, Carettochelys is unique or unusual among turtles in many facets of its morphology, ecology, and behavior. Populations in New Guinea are thought to be declining because of increased exploitation for meat and eggs for both domestic consumption and the international pet trade.