The Role of Trim58 in Erythropoiesis
Total Page:16
File Type:pdf, Size:1020Kb
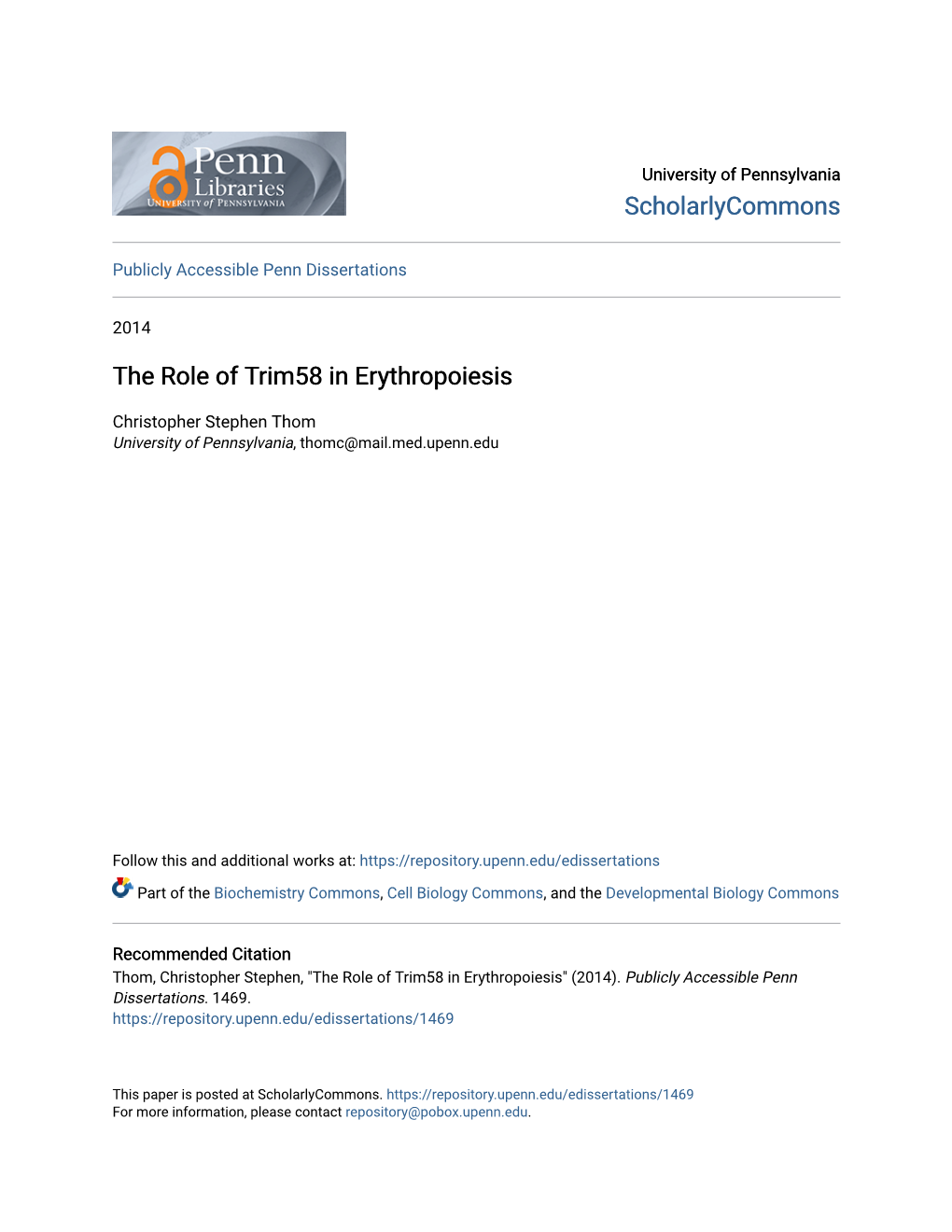
Load more
Recommended publications
-
The Genetic Program of Pancreatic Beta-Cell Replication in Vivo
Page 1 of 65 Diabetes The genetic program of pancreatic beta-cell replication in vivo Agnes Klochendler1, Inbal Caspi2, Noa Corem1, Maya Moran3, Oriel Friedlich1, Sharona Elgavish4, Yuval Nevo4, Aharon Helman1, Benjamin Glaser5, Amir Eden3, Shalev Itzkovitz2, Yuval Dor1,* 1Department of Developmental Biology and Cancer Research, The Institute for Medical Research Israel-Canada, The Hebrew University-Hadassah Medical School, Jerusalem 91120, Israel 2Department of Molecular Cell Biology, Weizmann Institute of Science, Rehovot, Israel. 3Department of Cell and Developmental Biology, The Silberman Institute of Life Sciences, The Hebrew University of Jerusalem, Jerusalem 91904, Israel 4Info-CORE, Bioinformatics Unit of the I-CORE Computation Center, The Hebrew University and Hadassah, The Institute for Medical Research Israel- Canada, The Hebrew University-Hadassah Medical School, Jerusalem 91120, Israel 5Endocrinology and Metabolism Service, Department of Internal Medicine, Hadassah-Hebrew University Medical Center, Jerusalem 91120, Israel *Correspondence: [email protected] Running title: The genetic program of pancreatic β-cell replication 1 Diabetes Publish Ahead of Print, published online March 18, 2016 Diabetes Page 2 of 65 Abstract The molecular program underlying infrequent replication of pancreatic beta- cells remains largely inaccessible. Using transgenic mice expressing GFP in cycling cells we sorted live, replicating beta-cells and determined their transcriptome. Replicating beta-cells upregulate hundreds of proliferation- related genes, along with many novel putative cell cycle components. Strikingly, genes involved in beta-cell functions, namely glucose sensing and insulin secretion were repressed. Further studies using single molecule RNA in situ hybridization revealed that in fact, replicating beta-cells double the amount of RNA for most genes, but this upregulation excludes genes involved in beta-cell function. -
PDF Download
NU153 Polyclonal Antibody Catalog No : YN0991 Reactivity : Human,Rat Applications : WB,ELISA Gene Name : NUP153 Protein Name : Nuclear pore complex protein Nup153 (153 kDa nucleoporin) (Nucleoporin Nup153) Human Gene Id : 9972 Human Swiss Prot P49790 No : Rat Swiss Prot No : P49791 Immunogen : Synthesized peptide derived from human protein . at AA range: 160-240 Specificity : NU153 Polyclonal Antibody detects endogenous levels of protein. Formulation : Liquid in PBS containing 50% glycerol, and 0.02% sodium azide. Source : Rabbit Dilution : WB 1:500-2000 ELISA 1:5000-20000 Purification : The antibody was affinity-purified from rabbit antiserum by affinity- chromatography using epitope-specific immunogen. Concentration : 1 mg/ml Storage Stability : -20°C/1 year Observed Band : 162 Background : nucleoporin 153(NUP153) Homo sapiens Nuclear pore complexes regulate the transport of macromolecules between the nucleus and cytoplasm. They are 1 / 2 composed of at least 100 different polypeptide subunits, many of which belong to the nucleoporin family. Nucleoporins are glycoproteins found in nuclear pores and contain characteristic pentapeptide XFXFG repeats as well as O-linked N- acetylglucosamine residues oriented towards the cytoplasm. The protein encoded by this gene has three distinct domains: a N-terminal region containing a pore targeting and an RNA-binding domain domain, a central region containing multiple zinc finger motifs, and a C-terminal region containing multiple XFXFG repeats. Alternative splicing results in multiple transcript -
C9orf72-Associated SMCR8 Protein Binds in the Ubiquitin Pathway and with Proteins Linked with Neurological Disease John L
Goodier et al. Acta Neuropathologica Communications (2020) 8:110 https://doi.org/10.1186/s40478-020-00982-x RESEARCH Open Access C9orf72-associated SMCR8 protein binds in the ubiquitin pathway and with proteins linked with neurological disease John L. Goodier1*, Alisha O. Soares1, Gavin C. Pereira1, Lauren R. DeVine2, Laura Sanchez3, Robert N. Cole2 and Jose Luis García-Pérez3,4 Abstract A pathogenic GGGCCC hexanucleotide expansion in the first intron/promoter region of the C9orf72 gene is the most common mutation associated with amyotrophic lateral sclerosis (ALS). The C9orf72 gene product forms a complex with SMCR8 (Smith-Magenis Syndrome Chromosome Region, Candidate 8) and WDR41 (WD Repeat domain 41) proteins. Recent studies have indicated roles for the complex in autophagy regulation, vesicle trafficking, and immune response in transgenic mice, however a direct connection with ALS etiology remains unclear. With the aim of increasing understanding of the multi-functional C9orf72-SMCR8-WDR41 complex, we determined by mass spectrometry analysis the proteins that directly associate with SMCR8. SMCR8 protein binds many components of the ubiquitin-proteasome system, and we demonstrate its poly-ubiquitination without obvious degradation. Evidence is also presented for localization of endogenous SMCR8 protein to cytoplasmic stress granules. However, in several cell lines we failed to reproduce previous observations that C9orf72 protein enters these granules. SMCR8 protein associates with many products of genes associated with various Mendelian neurological disorders in addition to ALS, implicating SMCR8-containing complexes in a range of neuropathologies. We reinforce previous observations that SMCR8 and C9orf72 protein levels are positively linked, and now show in vivo that SMCR8 protein levels are greatly reduced in brain tissues of C9orf72 gene expansion carrier individuals. -
Identification of Genomic Targets of Krüppel-Like Factor 9 in Mouse Hippocampal
Identification of Genomic Targets of Krüppel-like Factor 9 in Mouse Hippocampal Neurons: Evidence for a role in modulating peripheral circadian clocks by Joseph R. Knoedler A dissertation submitted in partial fulfillment of the requirements for the degree of Doctor of Philosophy (Neuroscience) in the University of Michigan 2016 Doctoral Committee: Professor Robert J. Denver, Chair Professor Daniel Goldman Professor Diane Robins Professor Audrey Seasholtz Associate Professor Bing Ye ©Joseph R. Knoedler All Rights Reserved 2016 To my parents, who never once questioned my decision to become the other kind of doctor, And to Lucy, who has pushed me to be a better person from day one. ii Acknowledgements I have a huge number of people to thank for having made it to this point, so in no particular order: -I would like to thank my adviser, Dr. Robert J. Denver, for his guidance, encouragement, and patience over the last seven years; his mentorship has been indispensable for my growth as a scientist -I would also like to thank my committee members, Drs. Audrey Seasholtz, Dan Goldman, Diane Robins and Bing Ye, for their constructive feedback and their willingness to meet in a frequently cold, windowless room across campus from where they work -I am hugely indebted to Pia Bagamasbad and Yasuhiro Kyono for teaching me almost everything I know about molecular biology and bioinformatics, and to Arasakumar Subramani for his tireless work during the home stretch to my dissertation -I am grateful for the Neuroscience Program leadership and staff, in particular -
Induction of Therapeutic Tissue Tolerance Foxp3 Expression Is
Downloaded from http://www.jimmunol.org/ by guest on October 2, 2021 is online at: average * The Journal of Immunology , 13 of which you can access for free at: 2012; 189:3947-3956; Prepublished online 17 from submission to initial decision 4 weeks from acceptance to publication September 2012; doi: 10.4049/jimmunol.1200449 http://www.jimmunol.org/content/189/8/3947 Foxp3 Expression Is Required for the Induction of Therapeutic Tissue Tolerance Frederico S. Regateiro, Ye Chen, Adrian R. Kendal, Robert Hilbrands, Elizabeth Adams, Stephen P. Cobbold, Jianbo Ma, Kristian G. Andersen, Alexander G. Betz, Mindy Zhang, Shruti Madhiwalla, Bruce Roberts, Herman Waldmann, Kathleen F. Nolan and Duncan Howie J Immunol cites 35 articles Submit online. Every submission reviewed by practicing scientists ? is published twice each month by Submit copyright permission requests at: http://www.aai.org/About/Publications/JI/copyright.html Receive free email-alerts when new articles cite this article. Sign up at: http://jimmunol.org/alerts http://jimmunol.org/subscription http://www.jimmunol.org/content/suppl/2012/09/17/jimmunol.120044 9.DC1 This article http://www.jimmunol.org/content/189/8/3947.full#ref-list-1 Information about subscribing to The JI No Triage! Fast Publication! Rapid Reviews! 30 days* Why • • • Material References Permissions Email Alerts Subscription Supplementary The Journal of Immunology The American Association of Immunologists, Inc., 1451 Rockville Pike, Suite 650, Rockville, MD 20852 Copyright © 2012 by The American Association of Immunologists, Inc. All rights reserved. Print ISSN: 0022-1767 Online ISSN: 1550-6606. This information is current as of October 2, 2021. -
Plasticity and the Impact of Increasing Temperature on a Tropical Ectotherm
Georgia Southern University Digital Commons@Georgia Southern Electronic Theses and Dissertations Graduate Studies, Jack N. Averitt College of Spring 2020 Plasticity and the Impact of Increasing Temperature on a Tropical Ectotherm Adam A. Rosso Follow this and additional works at: https://digitalcommons.georgiasouthern.edu/etd Part of the Bioinformatics Commons, Comparative and Evolutionary Physiology Commons, Evolution Commons, Integrative Biology Commons, Other Ecology and Evolutionary Biology Commons, and the Other Genetics and Genomics Commons Recommended Citation Rosso, Adam A., "Plasticity and the Impact of Increasing Temperature on a Tropical Ectotherm" (2020). Electronic Theses and Dissertations. 2069. https://digitalcommons.georgiasouthern.edu/etd/2069 This thesis (open access) is brought to you for free and open access by the Graduate Studies, Jack N. Averitt College of at Digital Commons@Georgia Southern. It has been accepted for inclusion in Electronic Theses and Dissertations by an authorized administrator of Digital Commons@Georgia Southern. For more information, please contact [email protected]. PLASTICITY AND THE IMPAPCT OF INCREASING TEMPERATURE ON A TROPICAL ECTOTHERM by ADAM A. ROSSO (Under the direction of Christian L. Cox) ABSTRACT Organisms may respond to climate change through behavior, genetic adaptation, and/or phenotypic plasticity. Tropical ectotherms are thought to be especially vulnerable to climate change because most have a narrow range of thermal tolerance while living close to their upper thermal tolerance limits. Additionally, many tropical species live in closed-canopy forests, which provide homogenous thermal landscapes that prevent behavioral compensation for stressfully warm temperatures. Finally, tropical ectotherms are thought to have decreased capacity for phenotypic plasticity because they have evolved in thermally stable environments. -
Flavone Effects on the Proteome and Transcriptome of Colonocytes in Vitro and in Vivo and Its Relevance for Cancer Prevention and Therapy
TECHNISCHE UNIVERSITÄT MÜNCHEN Lehrstuhl für Ernährungsphysiologie Flavone effects on the proteome and transcriptome of colonocytes in vitro and in vivo and its relevance for cancer prevention and therapy Isabel Winkelmann Vollständiger Abdruck der von der Fakultät Wissenschaftszentrum Weihenstephan für Ernährung, Landnutzung und Umwelt der Technischen Universität München zur Erlangung des akademischen Grades eines Doktors der Naturwissenschaften genehmigten Dissertation. Vorsitzender: Univ.-Prof. Dr. D. Haller Prüfer der Dissertation: 1. Univ.-Prof. Dr. H. Daniel 2. Univ.-Prof. Dr. U. Wenzel (Justus-Liebig-Universität Giessen) 3. Prof. Dr. E.C.M. Mariman (Maastricht University, Niederlande) schriftliche Beurteilung Die Dissertation wurde am 24.08.2009 bei der Technischen Universität München eingereicht und durch die Fakultät Wissenschaftszentrum Weihenstephan für Ernährung, Landnutzung und Umwelt am 25.11.2009 angenommen. Die Forschung ist immer auf dem Wege, nie am Ziel. (Adolf Pichler) Table of contents 1. Introduction .......................................................................................................... 1 1.1. Cancer and carcinogenesis .................................................................................. 2 1.2. Colorectal Cancer ............................................................................................... 3 1.2.1. Hereditary forms of CRC ........................................................................................ 4 1.2.2. Sporadic forms of CRC .......................................................................................... -
Downloaded from Ing Underlying Their Differentiation Into Specialized Cell Types
The University of Bradford Institutional Repository http://bradscholars.brad.ac.uk This work is made available online in accordance with publisher policies. Please refer to the repository record for this item and our Policy Document available from the repository home page for further information. To see the final version of this work please visit the publisher’s website. Access to the published online version may require a subscription. Link to publisher’s version: http://dx.doi.org/10.1083/jcb.201506065 Citation: Mardaryev AN, Liu B, Rapisarda V et al (2016) Cbx4 maintains the epithelial lineage identity and cell proliferation in the developing stratified epithelium. Journal of Cell Biology. 212(1): 77-89. Copyright statement: © 2016 Mardaryev et al. This article is distributed under the terms of a Creative Commons Attribution–Noncommercial–Share Alike 3.0 Unported license. Published December 28, 2015 JCB: Article Cbx4 maintains the epithelial lineage identity and cell proliferation in the developing stratified epithelium Andrei N. Mardaryev,1 Bo Liu,6 Valentina Rapisarda,1 Krzysztof Poterlowicz,1 Igor Malashchuk,1 Jana Rudolf,1 Andrey A. Sharov,2 Colin A. Jahoda,3 Michael Y. Fessing,1 Salvador A. Benitah,4,5 Guo-Liang Xu,6 and Vladimir A. Botchkarev1,2 1Centre for Skin Sciences, School of Life Sciences, University of Bradford, Yorkshire BD7 1DP, England, UK 2Department of Dermatology, Boston University School of Medicine, Boston, MA 02118 3School of Biological Sciences, University of Durham, Durham DH1 3LE, England, UK 4Institute for Research in Biomedicine, 08028 Barcelona, Spain 5Catalan Institution for Research and Advanced Studies, 08010 Barcelona, Spain 6The State Key Laboratory of Molecular Biology, Institute of Biochemistry and Cell Biology, Chinese Academy of Sciences, Shanghai 200031, China During development, multipotent progenitor cells establish lineage-specific programmers of gene activation and silenc- Downloaded from ing underlying their differentiation into specialized cell types. -
Transcriptomic Analysis of Patients with Tetralogy of Fallot Reveals the Effect of Chronic Hypoxia on Myocardial Gene Expression
Ghorbel et al Congenital Heart Disease Transcriptomic analysis of patients with tetralogy of Fallot reveals the effect of chronic hypoxia on myocardial gene expression Mohamed T. Ghorbel, PhD, Myriam Cherif, PhD, Emma Jenkins, PhD, Amir Mokhtari, MRCS, Damien Kenny, MRCPCH, Gianni D. Angelini, FRCS, and Massimo Caputo, MD Objectives: In cyanotic patients undergoing repair of heart defects, chronic hypoxia is thought to lead to greater susceptibility to ischemia and reoxygenation injury. We sought to find an explanation to such a hypothesis by investigating the cardiac gene expression in patients with tetralogy of Fallot undergoing cardiac surgery. CHD Methods: The myocardial gene profile was investigated in right ventricular biopsy specimens obtained from 20 patients with a diagnosis of cyanotic (n ¼ 11) or acyanotic (n ¼ 9) tetralogy of Fallot undergoing surgical repair. Oligonucleotide microarray analyses were performed on the samples, and the array results were validated with Western blotting and enzyme-linked immunosorbent assay. Results: Data revealed 795 differentially expressed genes in cyanotic versus acyanotic hearts, with 198 upregu- lated and 597 downregulated. Growth/morphogenesis, remodeling, and apoptosis emerged as dominant func- tional themes for the upregulated genes and included the apoptotic gene TRAIL (tumor necrosis factor–related apoptosis-inducing ligand), the remodeling factor OPN (osteopontin), and the mitochondrial function gene COX11 (cytochrome-c oxidase 11). In contrast, transcription, mitogen-activated protein kinase signaling, and contractile machinery were the dominant functional classes for the downregulated genes, which included the calcium-handling gene NCX1 (sodium-calcium exchanger). Protein levels of COX11, NCX1, OPN, and LYZ (ly- sozyme) in the myocardium followed the same pattern obtained by means of transcriptomics. -
List of Genes Deregulated in HDAC1 KO Vs WT
Yamaguchi_Suppl. Table 1 List of genes deregulated in HDAC1 KO vs WT Probe Set name fold change 1423327_at RIKEN cDNA 4930517K11 gene 201,6 1426231_at vitrin 33,4 1416368_at glutathione S-transferase, alpha 4 23,2 1435436_at Transcribed locus 20,1 1456379_x_at delta/notch-like EGF-related receptor 15,2 1418175_at vitamin D receptor 14,7 1455642_a_at tetraspanin 17 14,1 1436448_a_at prostaglandin-endoperoxide synthase 1 13,7 1423414_at prostaglandin-endoperoxide synthase 1 12,3 1416710_at transmembrane protein 35 11,8 1454780_at UDP-N-acetyl-alpha-D-galactosamine:polypeptide N-acetylgalactosaminyltransferase-like 4 10,9 1439231_at gb:BG228852 /DB_XREF=gi:12716356 /DB_XREF=ux62e05.x1 /CLONE=IMAGE:3514856 /FEA=EST /CNT=9 /TID=Mm.133155.1 /TIER=Stack10,3 /STK=8 /UG=Mm.133155 /UG_TITLE=ESTs 1416072_at CD34 antigen 10,2 1433529_at RIKEN cDNA E430002G05 gene 9,2 1416203_at aquaporin 1 8,6 1418949_at growth differentiation factor 15 8,3 1435955_at sialic acid binding Ig-like lectin 10 8,2 1416326_at cysteine-rich protein 1 (intestinal) 8,2 1448700_at G0/G1 switch gene 2 8,1 1416271_at PERP, TP53 apoptosis effector 8,0 1418176_at vitamin D receptor 7,8 1439789_at gb:BQ177189 /DB_XREF=gi:20352681 /DB_XREF=UI-M-DJ2-bwa-j-16-0-UI.s1 /CLONE=UI-M-DJ2-bwa-j-16-0-UI /FEA=EST /CNT=16 /TID=7,4 Mm.34073.1 /TIER=ConsEnd /STK=6 /UG=Mm.34073 /UG_TITLE=ESTs 1423627_at NAD(P)H dehydrogenase, quinone 1 7,3 1442174_at tetraspanin 18 7,3 1435945_a_at potassium intermediate/small conductance calcium-activated channel, subfamily N, member 4 6,9 1439362_at Transcribed -
Moderate Nucleoporin 133 Deficiency Leads to Glomerular Damage In
www.nature.com/scientificreports Corrected: Publisher Correction OPEN Moderate Nucleoporin 133 defciency leads to glomerular damage in zebrafsh Received: 28 June 2018 Chiara Cianciolo Cosentino1,4,5, Alessandro Berto2,3, Stéphane Pelletier2, Michelle Hari1, Accepted: 26 February 2019 Johannes Lofng4, Stephan C. F. Neuhauss1 & Valérie Doye2 Published online: 20 March 2019 Although structural nuclear pore proteins (nucleoporins) are seemingly required in every cell type to assemble a functional nuclear transport machinery, mutations or deregulation of a subset of them have been associated with specifc human hereditary diseases. In particular, previous genetic studies of patients with nephrotic syndrome identifed mutations in Nup107 that impaired the expression or the localization of its direct partner at nuclear pores, Nup133. In the present study, we characterized the zebrafsh nup133 orthologous gene and its expression pattern during larval development. Using a morpholino-mediated gene knockdown, we show that partial depletion of Nup133 in zebrafsh larvae leads to the formation of kidney cysts, a phenotype that can be rescued by co-injection of wild type mRNA. Analysis of diferent markers for tubular and glomerular development shows that the overall kidney development is not afected by nup133 knockdown. Likewise, no gross defect in nuclear pore complex assembly was observed in these nup133 morphants. On the other hand, nup133 downregulation results in proteinuria and moderate foot process efacement, mimicking some of the abnormalities typically featured by patients with nephrotic syndrome. These data indicate that nup133 is a new gene required for proper glomerular structure and function in zebrafsh. Efcient and regulated bidirectional transport between the cytoplasm and the nucleus is an essential process in all eukaryotic cells. -
UC San Diego UC San Diego Electronic Theses and Dissertations
UC San Diego UC San Diego Electronic Theses and Dissertations Title Study of NUP107 in human neurogenetics using zebrafish and induced-pluripotent stem cell models / Permalink https://escholarship.org/uc/item/9t70x07f Author Sotak, Bethany Nicole Publication Date 2012 Peer reviewed|Thesis/dissertation eScholarship.org Powered by the California Digital Library University of California UNIVERSITY OF CALIFORNIA, SAN DIEGO Study of NUP107 in human neurogenetics using zebrafish and induced-pluripotent stem cell models A dissertation submitted in partial satisfaction of the requirements for the degree Doctor of Philosophy in Biomedical Sciences by Bethany Nicole Sotak Committee in charge: Professor Joseph G. Gleeson, Chair Professor Lawrence S.B. Goldstein Professor Bruce A. Hamilton Professor Martin W. Hetzer Professor Alysson R. Muotri 2012 Copyright Bethany Nicole Sotak, 2012 All rights reserved. The Dissertation of Bethany Nicole Sotak is approved, and it is acceptable in quality and form for publication on microfilm and electronically: Chair University of California, San Diego 2012 iii DEDICATION I dedicate this dissertation to my love, Timothy Allen Ray, and friend Benjamin Horne. These men taught me how to experience life, but were both tragically taken from us too soon. Maintain the light. I cannot hear your laughter. I cannot see your smile. I wish that we could talk again, If only for a while. I know you’re watching over me Seeing everything I do. And though you’ll always be with me, I will always be missing you. You taught me that life is much too short And at any time could end. But know no matter where you are You will always be my best friend.