View of Entomology 47: 817-44
Total Page:16
File Type:pdf, Size:1020Kb
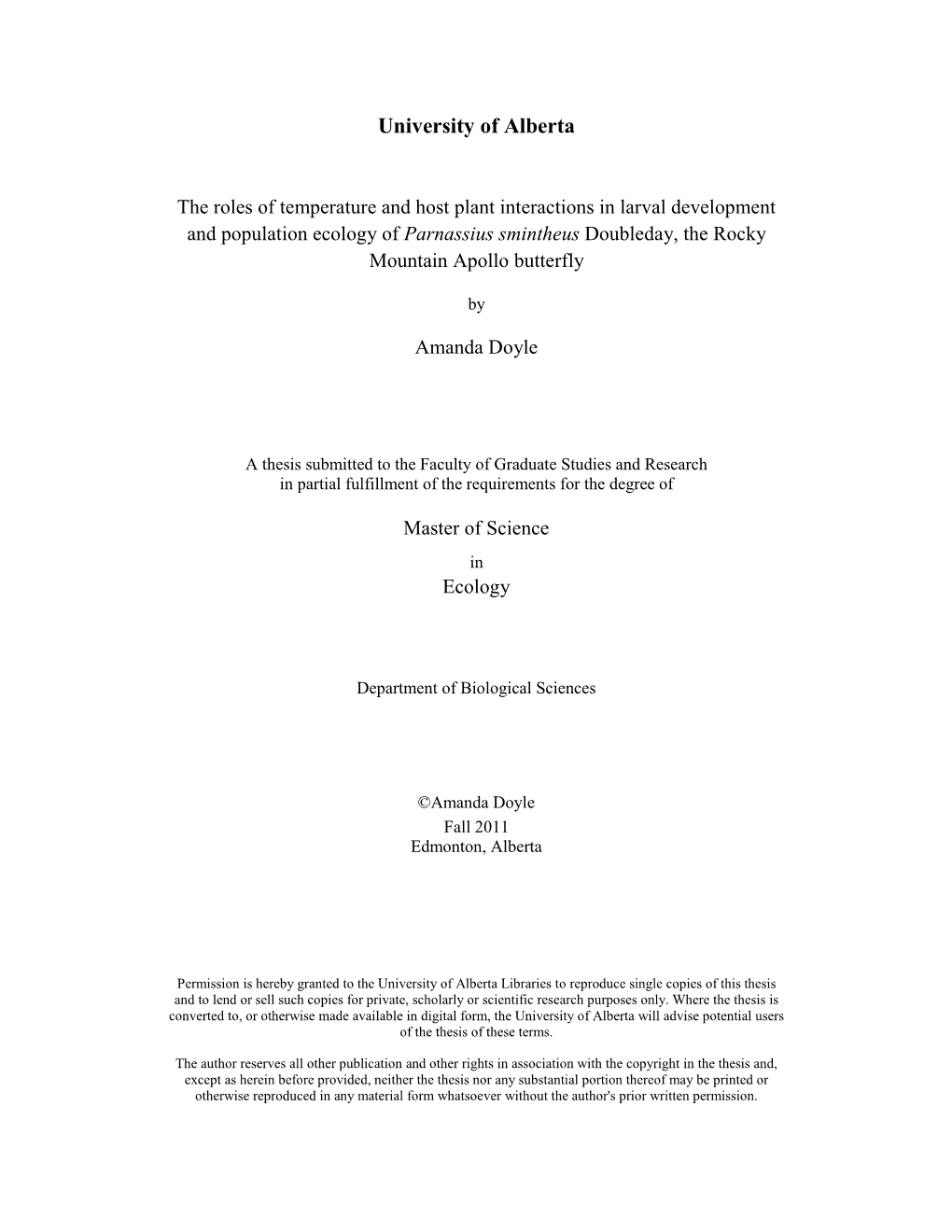
Load more
Recommended publications
-
Assessing Landscape Effects on Genetics and Dispersal of the Rocky Mountain Apollo Butterfly Arnassiusp Smintheus Using a Resistance Mapping Approach
Western University Scholarship@Western Electronic Thesis and Dissertation Repository 10-27-2017 10:30 AM Assessing Landscape Effects on Genetics and Dispersal of the Rocky Mountain Apollo Butterfly arnassiusP smintheus using a Resistance Mapping Approach Ning Chen The University of Western Ontario Supervisor Dr. Nusha Keyghobadi The University of Western Ontario Graduate Program in Biology A thesis submitted in partial fulfillment of the equirr ements for the degree in Master of Science © Ning Chen 2017 Follow this and additional works at: https://ir.lib.uwo.ca/etd Part of the Biology Commons Recommended Citation Chen, Ning, "Assessing Landscape Effects on Genetics and Dispersal of the Rocky Mountain Apollo Butterfly Parnassius smintheus using a Resistance Mapping Approach" (2017). Electronic Thesis and Dissertation Repository. 5058. https://ir.lib.uwo.ca/etd/5058 This Dissertation/Thesis is brought to you for free and open access by Scholarship@Western. It has been accepted for inclusion in Electronic Thesis and Dissertation Repository by an authorized administrator of Scholarship@Western. For more information, please contact [email protected]. Abstract Landscape variables that best explain genetic differentiation may not also best explain dispersal patterns, but many studies use genetic differentiation as a proxy for dispersal. I tested the effects of landscape on both genetic differentiation and dispersal in parallel, to explore whether landscape effects on genetic differentiation between populations and landscape effects on dispersal would be comparable in such contexts. I used circuit theory (Circuitscape) and least cost transect analysis to evaluate the effects of landscape on both movement and genetic differentiation of the butterfly, Parnassius smintheus, in the Jumpingpound Ridge study system. -
Large-Scale Movement Behavior in a Reintroduced Predator Population
doi: 10.1111/ecog.03030 41 126–139 ECOGRAPHY Research Large-scale movement behavior in a reintroduced predator population Frances E. Buderman, Mevin B. Hooten, Jacob S. Ivan and Tanya M. Shenk F. E. Buderman (http://orcid.org/0000-0001-9778-9906) ([email protected]) Dept of Fish, Wildlife, and Conservation Biology, Colorado State Univ., Fort Collins, CO, USA. – M. B. Hooten, U.S. Geological Survey, Colorado Cooperative Fish and Wildlife Research Unit, Dept of Fish, Wildlife, and Conservation Biology and Statistics, Colorado State Univ., Fort Collins, CO, USA, and Graduate Degree Program in Ecology, Colorado State Univ., Fort Collins, CO, USA. – J. S. Ivan, Colorado Parks and Wildlife, Fort Collins, CO, USA. – T. M. Shenk, National Park Service, Great Plains Cooperative Ecosystem Studies Unit, Univ. of Nebraska, Lincoln, NE, USA. Ecography Understanding movement behavior and identifying areas of landscape connectivity is 41: 126–139, 2018 critical for the conservation of many species. However, collecting fine-scale movement doi: 10.1111/ecog.03030 data can be prohibitively time consuming and costly, especially for rare or endangered species, whereas existing data sets may provide the best available information on animal Subject Editor: Timothy Keitt. movement. Contemporary movement models may not be an option for modeling Editor-in-Chief: Miguel Araújo. existing data due to low temporal resolution and large or unusual error structures, but Accepted 17 July 2017 inference can still be obtained using a functional movement modeling approach. We use a functional movement model to perform a population-level analysis of telemetry data collected during the reintroduction of Canada lynx to Colorado. -
Ours to Save: the Distribution, Status & Conservation Needs of Canada's Endemic Species
Ours to Save The distribution, status & conservation needs of Canada’s endemic species June 4, 2020 Version 1.0 Ours to Save: The distribution, status & conservation needs of Canada’s endemic species Additional information and updates to the report can be found at the project website: natureconservancy.ca/ourstosave Suggested citation: Enns, Amie, Dan Kraus and Andrea Hebb. 2020. Ours to save: the distribution, status and conservation needs of Canada’s endemic species. NatureServe Canada and Nature Conservancy of Canada. Report prepared by Amie Enns (NatureServe Canada) and Dan Kraus (Nature Conservancy of Canada). Mapping and analysis by Andrea Hebb (Nature Conservancy of Canada). Cover photo credits (l-r): Wood Bison, canadianosprey, iNaturalist; Yukon Draba, Sean Blaney, iNaturalist; Salt Marsh Copper, Colin Jones, iNaturalist About NatureServe Canada A registered Canadian charity, NatureServe Canada and its network of Canadian Conservation Data Centres (CDCs) work together and with other government and non-government organizations to develop, manage, and distribute authoritative knowledge regarding Canada’s plants, animals, and ecosystems. NatureServe Canada and the Canadian CDCs are members of the international NatureServe Network, spanning over 80 CDCs in the Americas. NatureServe Canada is the Canadian affiliate of NatureServe, based in Arlington, Virginia, which provides scientific and technical support to the international network. About the Nature Conservancy of Canada The Nature Conservancy of Canada (NCC) works to protect our country’s most precious natural places. Proudly Canadian, we empower people to safeguard the lands and waters that sustain life. Since 1962, NCC and its partners have helped to protect 14 million hectares (35 million acres), coast to coast to coast. -
Anthropogenic Threats to High-Altitude Parnassian Diversity Fabien Condamine, Felix Sperling
Anthropogenic threats to high-altitude parnassian diversity Fabien Condamine, Felix Sperling To cite this version: Fabien Condamine, Felix Sperling. Anthropogenic threats to high-altitude parnassian diversity. News of The Lepidopterists’ Society, 2018, 60, pp.94-99. hal-02323624 HAL Id: hal-02323624 https://hal.archives-ouvertes.fr/hal-02323624 Submitted on 23 Oct 2019 HAL is a multi-disciplinary open access L’archive ouverte pluridisciplinaire HAL, est archive for the deposit and dissemination of sci- destinée au dépôt et à la diffusion de documents entific research documents, whether they are pub- scientifiques de niveau recherche, publiés ou non, lished or not. The documents may come from émanant des établissements d’enseignement et de teaching and research institutions in France or recherche français ou étrangers, des laboratoires abroad, or from public or private research centers. publics ou privés. _______________________________________________________________________________________News of The Lepidopterists’ Society Volume 60, Number 2 Conservation Matters: Contributions from the Conservation Committee Anthropogenic threats to high-altitude parnassian diversity Fabien L. Condamine1 and Felix A.H. Sperling2 1CNRS, UMR 5554 Institut des Sciences de l’Evolution (Université de Montpellier), Place Eugène Bataillon, 34095 Montpellier, France [email protected], corresponding author 2Department of Biological Sciences, University of Alberta, Edmonton T6G 2E9, Alberta, Canada Introduction extents (Chen et al. 2011). However, this did not result in increases in range area because the area of land available Global mean annual temperatures increased by ~0.85° declines with increasing elevation. Accordingly, extinc- C between 1880 and 2012 and are likely to rise by an tion risk may increase long before species reach a summit, additional 1° C to 4° C by 2100 (Stocker et al. -
Yukon Butterflies a Guide to Yukon Butterflies
Wildlife Viewing Yukon butterflies A guide to Yukon butterflies Where to find them Currently, about 91 species of butterflies, representing five families, are known from Yukon, but scientists expect to discover more. Finding butterflies in Yukon is easy. Just look in any natural, open area on a warm, sunny day. Two excellent butterfly viewing spots are Keno Hill and the Blackstone Uplands. Pick up Yukon’s Wildlife Viewing Guide to find these and other wildlife viewing hotspots. Visitors follow an old mining road Viewing tips to explore the alpine on top of Keno Hill. This booklet will help you view and identify some of the more common butterflies, and a few distinctive but less common species. Additional species are mentioned but not illustrated. In some cases, © Government of Yukon 2019 you will need a detailed book, such as , ISBN 978-1-55362-862-2 The Butterflies of Canada to identify the exact species that you have seen. All photos by Crispin Guppy except as follows: In the Alpine (p.ii) Some Yukon butterflies, by Ryan Agar; Cerisy’s Sphynx moth (p.2) by Sara Nielsen; Anicia such as the large swallowtails, Checkerspot (p.2) by Bruce Bennett; swallowtails (p.3) by Bruce are bright to advertise their Bennett; Freija Fritillary (p.12) by Sonja Stange; Gallium Sphinx presence to mates. Others are caterpillar (p.19) by William Kleeden (www.yukonexplorer.com); coloured in dull earth tones Butterfly hike at Keno (p.21) by Peter Long; Alpine Interpretive that allow them to hide from bird Centre (p.22) by Bruce Bennett. -
Rationales for Animal Species Considered for Designation As Species of Conservation Concern Inyo National Forest
Rationales for Animal Species Considered for Designation as Species of Conservation Concern Inyo National Forest Prepared by: Wildlife Biologists and Natural Resources Specialist Regional Office, Inyo National Forest, and Washington Office Enterprise Program for: Inyo National Forest August 2018 1 In accordance with Federal civil rights law and U.S. Department of Agriculture (USDA) civil rights regulations and policies, the USDA, its Agencies, offices, and employees, and institutions participating in or administering USDA programs are prohibited from discriminating based on race, color, national origin, religion, sex, gender identity (including gender expression), sexual orientation, disability, age, marital status, family/parental status, income derived from a public assistance program, political beliefs, or reprisal or retaliation for prior civil rights activity, in any program or activity conducted or funded by USDA (not all bases apply to all programs). Remedies and complaint filing deadlines vary by program or incident. Persons with disabilities who require alternative means of communication for program information (e.g., Braille, large print, audiotape, American Sign Language, etc.) should contact the responsible Agency or USDA’s TARGET Center at (202) 720-2600 (voice and TTY) or contact USDA through the Federal Relay Service at (800) 877-8339. Additionally, program information may be made available in languages other than English. To file a program discrimination complaint, complete the USDA Program Discrimination Complaint Form, AD-3027, found online at http://www.ascr.usda.gov/complaint_filing_cust.html and at any USDA office or write a letter addressed to USDA and provide in the letter all of the information requested in the form. To request a copy of the complaint form, call (866) 632-9992. -
A SKELETON CHECKLIST of the BUTTERFLIES of the UNITED STATES and CANADA Preparatory to Publication of the Catalogue Jonathan P
A SKELETON CHECKLIST OF THE BUTTERFLIES OF THE UNITED STATES AND CANADA Preparatory to publication of the Catalogue © Jonathan P. Pelham August 2006 Superfamily HESPERIOIDEA Latreille, 1809 Family Hesperiidae Latreille, 1809 Subfamily Eudaminae Mabille, 1877 PHOCIDES Hübner, [1819] = Erycides Hübner, [1819] = Dysenius Scudder, 1872 *1. Phocides pigmalion (Cramer, 1779) = tenuistriga Mabille & Boullet, 1912 a. Phocides pigmalion okeechobee (Worthington, 1881) 2. Phocides belus (Godman and Salvin, 1890) *3. Phocides polybius (Fabricius, 1793) =‡palemon (Cramer, 1777) Homonym = cruentus Hübner, [1819] = palaemonides Röber, 1925 = ab. ‡"gunderi" R. C. Williams & Bell, 1931 a. Phocides polybius lilea (Reakirt, [1867]) = albicilla (Herrich-Schäffer, 1869) = socius (Butler & Druce, 1872) =‡cruentus (Scudder, 1872) Homonym = sanguinea (Scudder, 1872) = imbreus (Plötz, 1879) = spurius (Mabille, 1880) = decolor (Mabille, 1880) = albiciliata Röber, 1925 PROTEIDES Hübner, [1819] = Dicranaspis Mabille, [1879] 4. Proteides mercurius (Fabricius, 1787) a. Proteides mercurius mercurius (Fabricius, 1787) =‡idas (Cramer, 1779) Homonym b. Proteides mercurius sanantonio (Lucas, 1857) EPARGYREUS Hübner, [1819] = Eridamus Burmeister, 1875 5. Epargyreus zestos (Geyer, 1832) a. Epargyreus zestos zestos (Geyer, 1832) = oberon (Worthington, 1881) = arsaces Mabille, 1903 6. Epargyreus clarus (Cramer, 1775) a. Epargyreus clarus clarus (Cramer, 1775) =‡tityrus (Fabricius, 1775) Homonym = argentosus Hayward, 1933 = argenteola (Matsumura, 1940) = ab. ‡"obliteratus" -
Effects of Experimental Population Extinction for the Spatial Population Dynamics of the Butterfl Y Parnassius Smintheus
Oikos 119: 1961–1969, 2010 doi: 10.1111/j.1600-0706.2010.18666.x © 2010 e Authors. Oikos © 2010 Nordic Society Oikos Subject Editor: Daniel Gruner. Accepted 26 April 2010 Effects of experimental population extinction for the spatial population dynamics of the butterfl y Parnassius smintheus Stephen F. Matter and Jens Roland S. F. Matter ([email protected]), Dept of Biological Sciences, Univ. of Cincinnati, Cincinnati, OH 45220, USA. – J. Roland, Dept of Biological Sciences, Univ. of Alberta, Edmonton, AB T5K 1X4, Canada. While many studies have examined factors potentially impacting the rate of local population extinction, few experimental studies have examined the consequences of extinction for spatial population dynamics. Here we report results from a large- scale, long-term experiment examining the e ects of local population extinction for the dynamics of surrounding popula- tions. From 2001 – 2008 we removed all adult butterfl ies from two large, neighboring populations within a system of 17 subpopulations of the Rocky Mountain Apollo butterfl y, Parnassius smintheus . Surrounding populations were monitored using individual, mark–recapture methods. We found that population removal decreased immigration to surrounding populations in proportion to their connectivity to the removed populations. Correspondingly, within-generation popula- tion abundance declined. Despite these e ects, we saw little consistent impact between generations. e extinction rates of surrounding populations were una ected and local population growth was not consistently reduced by the lack of immigra- tion. e broader results show that immigration a ects local abundance within generations, but dynamics are mediated by density-dependence within populations and by broader density-independent factors acting between generations. -
The Coleopteran Fauna of Sultan Creek-Molas Lake Area with Special Emphasis on Carabidae and How the Geological Bedrock Influenc
THE COLEOPTERAN FAUNA OF SULTAN CREEK-MOLAS LAKE AREA WITH SPECIAL EMPHASIS ON CARABIDAE AND HOW THE GEOLOGICAL BEDROCK INFLUENCES BIODIVERSITY AND COMMUNITY STRUCTURE IN THE SAN JUAN MOUNTAINS, SAN JUAN COUNTY, COLORADO Melanie L. Bergolc A Dissertation Submitted to the Graduate College of Bowling Green State University in partial fulfillment of the requirements for the degree of DOCTOR OF PHILOSOPHY August 2009 Committee: Daniel Pavuk, Advisor Kurt Panter Graduate Faculty Representative Jeff Holland Rex Lowe Moira van Staaden © 2009 Melanie L. Bergolc All Rights Reserved iii ABSTRACT Daniel Pavuk, Advisor Few studies have been performed on coleopteran (beetle) biodiversity in mountain ecosystems and relating them to multiple environmental factors. None of the studies have examined geologic influences on beetle communities. Little coleopteran research has been performed in the Colorado Rocky Mountains. The main objectives of this study were to catalog the coleopteran fauna of a subalpine meadow in the San Juan Mountains of Colorado and investigate the role geology had in the community structure of the Carabidae (ground beetles). The study site, a 160,000 m2 plot, was located near Sultan Creek and Molas Lake in San Juan County, Colorado. Five sites were in each bedrock formation: Molas, Elbert, and Ouray-Leadville. Insects were collected via pitfall trapping in 2006 and 2007, and identified by comparison with museum specimens, museum and insect identification websites, and by taxonomic experts. Biological and physical factors were recorded for each site: detritus cover and weight, plant cover and height, plant species richness, aspect, elevation, slope, soil temperature, pH, moisture, and compressive strength, and sediment size distribution. -
Sentinels on the Wing: the Status and Conservation of Butterflies in Canada
Sentinels on the Wing The Status and Conservation of Butterflies in Canada Peter W. Hall Foreword In Canada, our ties to the land are strong and deep. Whether we have viewed the coasts of British Columbia or Cape Breton, experienced the beauty of the Arctic tundra, paddled on rivers through our sweeping boreal forests, heard the wind in the prairies, watched caribou swim the rivers of northern Labrador, or searched for song birds in the hardwood forests of south eastern Canada, we all call Canada our home and native land. Perhaps because Canada’s landscapes are extensive and cover a broad range of diverse natural systems, it is easy for us to assume the health of our important natural spaces and the species they contain. Our country seems so vast compared to the number of Canadians that it is difficult for us to imagine humans could have any lasting effect on nature. Yet emerging science demonstrates that our natural systems and the species they contain are increas- ingly at risk. While the story is by no means complete, key indicator species demonstrate that Canada’s natural legacy is under pressure from a number of sources, such as the conversion of lands for human uses, the release of toxic chemicals, the introduction of new, invasive species or the further spread of natural pests, and a rapidly changing climate. These changes are hitting home and, with the globalization and expansion of human activities, it is clear the pace of change is accelerating. While their flights of fancy may seem insignificant, butterflies are sentinels or early indicators of this change, and can act as important messengers to raise awareness. -
Cyanogenesis in Arthropods: from Chemical Warfare to Nuptial Gifts
Cyanogenesis in arthropods from chemical warfare to nuptial gifts Zagrobelny, Mika; Pinheiro de Castro, Érika Cristina; Møller, Birger Lindberg; Bak, Søren Published in: Insects DOI: 10.3390/insects9020051 Publication date: 2018 Document version Publisher's PDF, also known as Version of record Citation for published version (APA): Zagrobelny, M., Pinheiro de Castro, É. C., Møller, B. L., & Bak, S. (2018). Cyanogenesis in arthropods: from chemical warfare to nuptial gifts. Insects, 9(2), [51]. https://doi.org/10.3390/insects9020051 Download date: 30. sep.. 2021 insects Review Cyanogenesis in Arthropods: From Chemical Warfare to Nuptial Gifts Mika Zagrobelny 1,* ID , Érika Cristina Pinheiro de Castro 2, Birger Lindberg Møller 1,3 ID and Søren Bak 1 ID 1 Plant Biochemistry Laboratory, Department of Plant and Environmental Sciences, University of Copenhagen, 1871 Frederiksberg C, Denmark; [email protected] (B.L.M.); [email protected] (S.B.) 2 Department of Ecology, Federal University of Rio Grande do Norte, Natal-RN, 59078-900, Brazil; [email protected] 3 VILLUM Center for Plant Plasticity, University of Copenhagen, 1871 Frederiksberg C, Denmark * Correspondence: [email protected] Received: 7 March 2018; Accepted: 24 April 2018; Published: 3 May 2018 Abstract: Chemical defences are key components in insect–plant interactions, as insects continuously learn to overcome plant defence systems by, e.g., detoxification, excretion or sequestration. Cyanogenic glucosides are natural products widespread in the plant kingdom, and also known to be present in arthropods. They are stabilised by a glucoside linkage, which is hydrolysed by the action of β-glucosidase enzymes, resulting in the release of toxic hydrogen cyanide and deterrent aldehydes or ketones. -
J91J Field Season Summary
Number 2 NEWS 15 March, 1972 of the LEPIDOPTERISTS' SOCIETY Editorial Committee of the NEWS . ..•. EDITOR: Dr. Charles V. Covell, Jr., Dept. of Biology, Univ. of Louisville, Louisville, Ky., USA, 40208 ASSOC. EDITOR: Dr. Paul A. Opler, Div. of Entomology, 201 Wellman Hall, Univ. of California, Berkeley, California, USA, 94720 J. Donald Eff G. Hesselbarth K. W. Philip Thomas C. Emmel Robert L. Langston F. W. Preston Lloyd M. Martin H. A. Freeman G. W. Rawson F. Bryant Mather L. Paul Grey M. C. Nielsen E. C. Welling M. John Heath E. J. Newcomer R. S. Wilkinson J91J Field Season Summary Here is the summary of last year's collecting, in time, I hope, to aid you with your collecting plans for 1972. It is varied in coverage and detail, reflecting the reporting differences among contributors and coordinators. The purpose of this Summary is, as I understand it, to give our members al1'opportunity to report collecting conditions and productive localities, along with interesting occurrences or lack of familiar species, in the areas they have covered. It is not meant to be the vehicle of publication for "permanent record" of important faunistic data, although many state records and life history notes appear informally here. There is, however, a tendency of faunal students to cite records in past Summaries. Thus the Summary is, in reality, somewhat more than it was originally intended to be. We try to include something from each contributor, and encourage all who wish to send in brief reports to be included. The 1971 season appears to have been average to somewhat below average in North America, with cold win ter, spring and summer in much of the West, combined with drought in the summer.