Soil Stabilisatizion for DNA Metabarcoding of Plants and Fungi
Total Page:16
File Type:pdf, Size:1020Kb
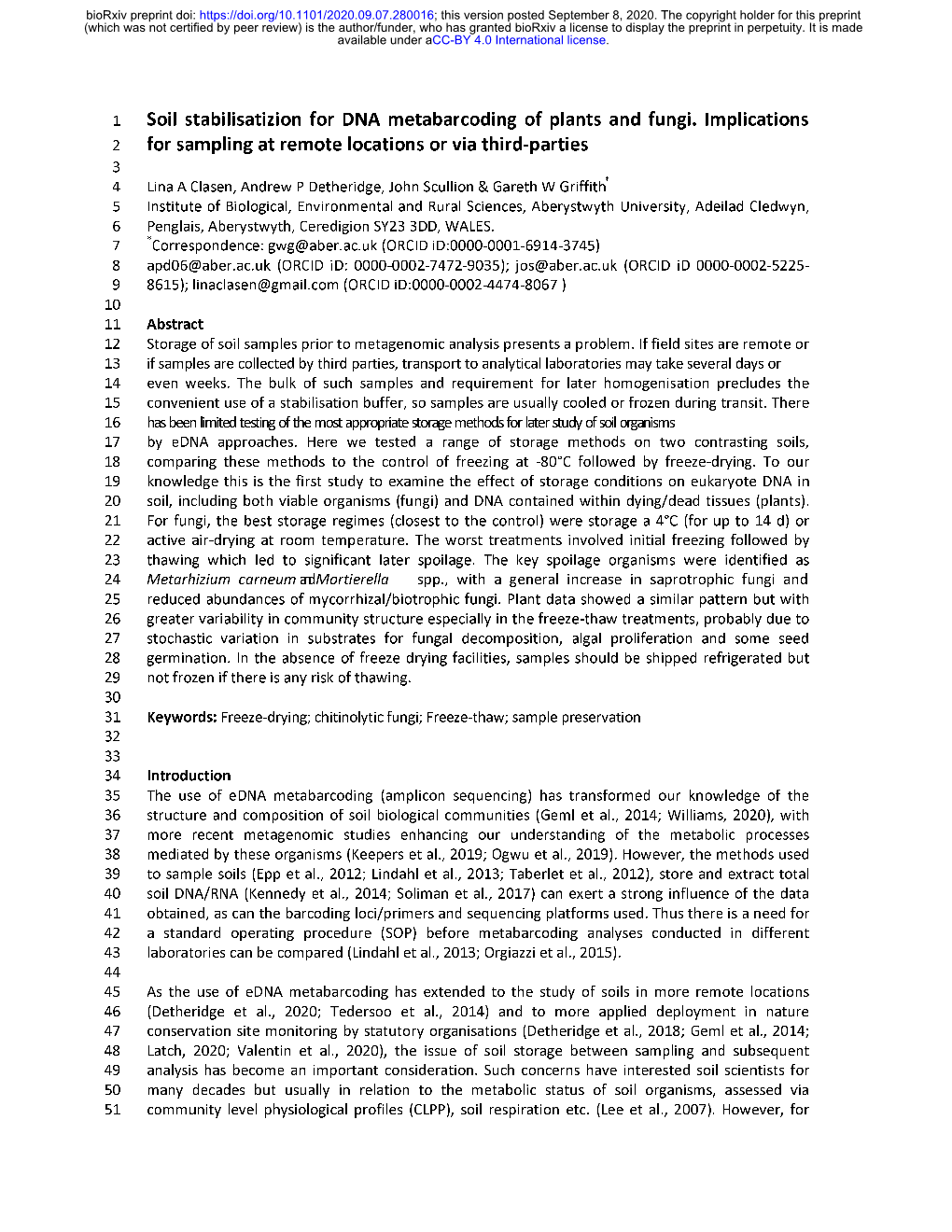
Load more
Recommended publications
-
Systematics, Barcoding and Ecology of Fungi from Waxcap Grasslands in Britain
Project report to DEFRA 1: 3 December 2010 Systematics, barcoding and ecology of fungi from waxcap grasslands in Britain Collaborations An important component of the project is to bring together professional scientists and specialist volunteers to contribute specimens and ecological data, and later on to explore ways of improving the existing recording and monitoring schemes. The project will provide valuable data to aid decision-making for conservation management, for example designation of SSSIs and establishment of red data lists. The first action was therefore to publicize the project to potential collaborators, and emails and verbal communications took place both directly with known collectors/recording groups and through the British Mycological Society. Excluding those named in the project application, 33 individuals/recording groups have provided specimens and/or identification data. Our initial request was for all species of Hygrocybe and Geoglossaceae. While a number of these are common and widespread, there are concerns that existing species concepts are too broad and are masking cryptic taxa. Along with specimens that we have collected ourselves, we have received a total of 213 collections belonging to 34 species/varieties of Hygrocybe and four species of Geoglossaceae. The collections have come from 27 different vice-counties in England, Wales and Scotland. Much of the field season was poor for waxcap species, although a number of species fruited abnormally late in the year. With this in mind, we are very happy with the response we have received from field workers, and there has been a widespread welcome for our project. We are confident that our field collaborators will continue to support our work throughout the project period and beyond. -
As I Mentioned in the Spring Edition, the Aim Is to Produce Our News
Some thoughts on the HFSG News Sheet President & Recorder: Ted Blackwell As I mentioned in the Spring issue News Sheet, the tel. 01568 780480; aim is now to try to produce an issue twice per e-mail: [email protected] year. This would seem to work out as publications in: Chair & Secretary: Sheila Spence . late April/early May, covering the September – tel. 01531 631736; February forays; e-mail: [email protected] . late September/early October, covering the March – August forays. Treasurer: Ray Bray tel. 01531 670301 Both the last issue and this current one rely very e-mail: [email protected] heavily on contributions from Ted and Ray, to whom I am most grateful for their willing(?) submission to blackmail! It would be pleasing, CHAIRMAN’S MESSAGE though, if our News Sheet reflected more widely the talents, as well as the wishes, of the whole Group The Spring foraying season started with great and I hope that future issues will increasingly do enthusiasm: it was great to be out in the woods this. again! My first season as Chairman has gone smoothly, due to the great support of members - in It is, I think, desirable to keep both the size and particular Shelly and Mike, for leading the foray to content of these News Sheets as flexible as Netherwood and, of course, Ted for his continuing, possible. However, each issue will, hopefully, unstinting help and guidance. We have had some contain, as a common denominator: really good forays so far: thank you, Ted, for giving us all the info. on the exciting finds we have made. -
And Interspecific Hybridiation in Agaric Fungi
Mycologia, 105(6), 2013, pp. 1577–1594. DOI: 10.3852/13-041 # 2013 by The Mycological Society of America, Lawrence, KS 66044-8897 Evolutionary consequences of putative intra- and interspecific hybridization in agaric fungi Karen W. Hughes1 to determine the outcome of hybridization events. Ronald H. Petersen Within Armillaria mellea and Amanita citrina f. Ecology and Evolutionary Biology, University of lavendula, we found evidence of interbreeding and Tennessee, Knoxville, Tennessee 37996-1100 recombination. Within G. dichrous and H. flavescens/ D. Jean Lodge chlorophana, hybrids were identified but there was Center for Forest Mycology Research, USDA-Forest no evidence for F2 or higher progeny in natural Service, Northern Research Station, Box 137, Luquillo, populations suggesting that the hybrid fruitbodies Puerto Rico 00773-1377 might be an evolutionary dead end and that the Sarah E. Bergemann genetically divergent Mendelian populations from which they were derived are, in fact, different species. Middle Tennessee State University, Department of Biology, PO Box 60, Murfreesboro Tennessee 37132 The association between ITS haplotype divergence of less than 5% (Armillaria mellea 5 2.6% excluding Kendra Baumgartner gaps; Amanita citrina f. lavendula 5 3.3%) with the USDA-Agricultural Research Service, Department of presence of putative recombinants and greater than Plant Pathology, University of California, Davis, California 95616 5% (Gymnopus dichrous 5 5.7%; Hygrocybe flavescens/ chlorophana 5 14.1%) with apparent failure of F1 2 Rodham E. Tulloss hybrids to produce F2 or higher progeny in popula- PO Box 57, Roosevelt, New Jersey 08555-0057 tions may suggest a correlation between genetic Edgar Lickey distance and reproductive isolation. -
Habitat Specificity of Selected Grassland Fungi in Norway John Bjarne Jordal1, Marianne Evju2, Geir Gaarder3 1Biolog J.B
Habitat specificity of selected grassland fungi in Norway John Bjarne Jordal1, Marianne Evju2, Geir Gaarder3 1Biolog J.B. Jordal, Auragata 3, NO-6600 Sunndalsøra 2Norwegian Institute for Nature Research, Gaustadalléen 21, NO-0349 Oslo 3Miljøfaglig Utredning, Gunnars veg 10, NO-6610 Tingvoll Corresponding author: er undersøkt når det gjelder habitatspesifisitet. [email protected] 70 taksa (53%) har mindre enn 10% av sine funn i skog, mens 23 (17%) har mer enn 20% Norsk tittel: Habitatspesifisitet hos utvalgte av funnene i skog. De som har høyest frekvens beitemarkssopp i Norge i skog i Norge er for det meste også vanligst i skog i Sverige. Jordal JB, Evju M, Gaarder G, 2016. Habitat specificity of selected grassland fungi in ABSTRACT Norway. Agarica 2016, vol. 37: 5-32. 132 taxa of fungi regularly found in semi- natural grasslands from the genera Camaro- KEY WORDS phyllopsis, Clavaria, Clavulinopsis, Dermo- Grassland fungi, seminatural grasslands, loma, Entoloma, Geoglossum, Hygrocybe, forests, other habitats, Norway Microglossum, Porpoloma, Ramariopsis and Trichoglossum were selected. Their habitat NØKKELORD specificity was investigated based on 39818 Beitemarkssopp, seminaturlige enger, skog, records from Norway. Approximately 80% of andre habitater, Norge the records were from seminatural grasslands, ca. 10% from other open habitats like parks, SAMMENDRAG gardens and road verges, rich fens, coastal 132 taksa av sopp med regelmessig fore- heaths, open rocks with shallow soil, waterfall komst i seminaturlig eng av slektene Camaro- meadows, scree meadows and alpine habitats, phyllopsis, Clavaria, Clavulinopsis, Dermo- while 13% were found in different forest loma, Entoloma, Geoglossum, Hygrocybe, types (some records had more than one Microglossum, Porpoloma, Ramariopsis og habitat type, the sum therefore exceeds 100%). -
Blyttia Norsk Botanisk Forenings Tidsskrift Journal of the Norwegian Botanical Society
BLYTTIA NORSK BOTANISK FORENINGS TIDSSKRIFT JOURNAL OF THE NORWEGIAN BOTANICAL SOCIETY 2/2000 ÅRGANG 58 ISSN 0006-5269 http://www.toyen.uio.no/botanisk/nbf/blyttia/ BLYTTIAGALLERIET NBF-medlem Aage Pedersen i Støvring i Nord-Jylland har sendt inn en samling bilder fra Bårdsengbekken i Øyer. Han skriver: “Med hjælp af Blyttia bind 41 hefte 2 (Berg 1983) genfandt jeg i 1995 skogranke Clematis alpina ssp. sibirica i Bårdsengkløften. Over 25 år tidligere havde jeg set planten ovenfor Tretten efter anvisning af købmand Nustad i Tretten og et gensyn med skogranke – og behørig fotografering – stod meget højt på min ønskeliste. På min vej op i kløften var der gode botaniske grunde til talrige hvil, og efter 800 m henad og 300 m opad fandt jeg igen en plads til min rygsækstol. Jeg vendte mig om, satte mig ned og så, at jeg var gået lige forbi en stor og meget flot bevoksning af skogranke. Huldregras Cinna latifolia og andet godt måtte komme i anden række, men bidrog selvklart til en perfekt dag langs Bårdsengbekken.” Flueblomst Ophrys insectifera. Foto: Arne Kildebo BLYTTIA i dette nummer: NORSK En ny og BOTANISK FORENINGS vakker TIDSSKRIFT vokssopp for Norge er funnet i Utgitt uten støtte fra Norges Forskningsråd. Ålesund. Arten, som Artikler i Blyttia er indeksert/abstrahert i: Bibliography of forfatterne foreslår å Agriculture, Biological Abstracts, Life Sciences Col- kalle rosavokssopp, hø- lection, Norske Tidsskriftartikler og Selected Water rer til blant en gruppe Resources Abstracts. sopparter knyttet til en- ger med relativt lang Redaktør: Jan Wesenberg kontinuitet. Se John I redaksjonen: Trond Grøstad, Klaus Høiland, Tor H. -
OF UKRAINE © Chvikov V., Prylutskyi O
Біорізноманіття, екологія та експериментальна біологія, 2020, №2 MYCOLOGY UDC 582.284 (477) Prylutskyi O. https://orcid.org/0000-0001-5730-517X Chvikov V. https://orcid.org/0000-0001-5297-3996 ANNOTATED CHECKLIST OF HYGROPHORACEAE (AGARICALES, BASIDIOMYCOTA) OF UKRAINE © Chvikov V., Prylutskyi O. V.N. Karazin Kharkiv National University [email protected] , [email protected] https://doi.org/10.34142/2708-5848.2020.22.2.01 Hygrophoraceae is a family within Agaricales, which comprises 26 genera and approximately 690 agaricoid species, including ectomycorrhizal, lichen-forming, bryophilous, humus and litter decomposing fungi. Some of these species especially those from genera Cuphophyllus, Hygrocybe, Neohygrocybe and Porpolomopsis are associated with natural grasslands and show extreme sensitivity to the presence of nitrogen-containing fertilizers in their substrate. This makes them indicative species of grasslands of high conservation value. While casual observations of Hygrophoraceae of Ukraine were incorporated in studies of agaricoid fungi as a whole, this family has never been in the focus of special research. Previously accumulated data on the diversity of Hygrophoraceae in Ukraine must be aggregated and revised. We have summarized all available data on the occurrences of Hygrophoraceae in Ukraine, including published papers, open databases, citizen science observations, and the previously unpublished original collection materials. Also, we provide an original description of the rare European species Haasiella venustissima (Fr.) Kotl. & Pouzar ex Chiaffi & Surault, which is reported for the first time from the territory of Ukraine. The resulting checklist of Hygrophoraceae of Ukraine includes 66 species. Leading genera are Hygrophorus (22 species), Hygrocybe (17) and Arrhenia (10); 5 species among them (Hygrocybe punicea, Hyrgocybe splendidissima, Neohygrocybe nitrata, Neohygrocybe ovina, Porpolomopsis calyptriformis) are threatened and according to “IUCN Red List” considered “Vulnerable”. -
Ingleborough NNR
Ingleborough NNR Waxcap Grassland Survey January 2018 Ref: NEFU2017-243 Author: A.McLay Checked: Dr A.Jukes Natural England Field Unit CONTENTS Background 3 Introduction 3 Survey methodology 4 Survey results 4 Site evaluation 15 Recommendations 21 References 22 Appendices - Appendix 1. Location of units 23 - Appendix 2. List of non-CHEGD spp. 25 - Appendix 3. Grid locations for species 26 Appendix 4. Additional photographs 27 2 Introduction The term “waxcap grassland” was coined relatively recently to describe semi-natural grassland habitats containing distinctive assemblages of fungi, including waxcaps. Waxcaps are a group of fungi characterised by having thick waxy brittle gills, often bright colours and a preference for growing in unfertilised pastures or lawns. A waxcap grassland also frequently contains representatives of several other key grassland fungi groups, of which the fairy clubs (Clavariaceae family), earthtongues (Geoglossaceae) and pinkgills belonging to the genus Entoloma are the most prominent. Collectively these groups are often referred to as the CHEGD fungi, an acronymn derived from their initials. Additional grassland fungi representatives from the genera Dermoloma, Porpoloma and Camarophyllopsis are also included as honorary CHEGD fungi. The common factor linking these fungi groups is their requirement for nutrient-poor soil types, i.e. agriculturally unimproved grasslands. Such grasslands have usually received little or no input from modern agricultural nitrogen-based fertilisers and frequently support a semi-natural sward with fine-leaved grass species such as Festuca ovina, Agrostis capillaris and Anthoxanthum odoratum. A well-developed moss layer is almost always present and usually contains the widespread grassland moss species Rhytidiadelphus squarrosus. Waxcap grasslands usually have a well-grazed sward that is maintained by regular livestock browsing or frequent mowing. -
Suomen Helttasienten Ja Tattien Ekologia, Levinneisyys Ja Uhanalaisuus
Suomen ympäristö 769 LUONTO JA LUONNONVARAT Pertti Salo, Tuomo Niemelä, Ulla Nummela-Salo ja Esteri Ohenoja (toim.) Suomen helttasienten ja tattien ekologia, levinneisyys ja uhanalaisuus .......................... SUOMEN YMPÄRISTÖKESKUS Suomen ympäristö 769 Pertti Salo, Tuomo Niemelä, Ulla Nummela-Salo ja Esteri Ohenoja (toim.) Suomen helttasienten ja tattien ekologia, levinneisyys ja uhanalaisuus SUOMEN YMPÄRISTÖKESKUS Viittausohje Viitatessa tämän raportin lukuihin, käytetään lukujen otsikoita ja lukujen kirjoittajien nimiä: Esim. luku 5.2: Kytövuori, I., Nummela-Salo, U., Ohenoja, E., Salo, P. & Vauras, J. 2005: Helttasienten ja tattien levinneisyystaulukko. Julk.: Salo, P., Niemelä, T., Nummela-Salo, U. & Ohenoja, E. (toim.). Suomen helttasienten ja tattien ekologia, levin- neisyys ja uhanalaisuus. Suomen ympäristökeskus, Helsinki. Suomen ympäristö 769. Ss. 109-224. Recommended citation E.g. chapter 5.2: Kytövuori, I., Nummela-Salo, U., Ohenoja, E., Salo, P. & Vauras, J. 2005: Helttasienten ja tattien levinneisyystaulukko. Distribution table of agarics and boletes in Finland. Publ.: Salo, P., Niemelä, T., Nummela- Salo, U. & Ohenoja, E. (eds.). Suomen helttasienten ja tattien ekologia, levinneisyys ja uhanalaisuus. Suomen ympäristökeskus, Helsinki. Suomen ympäristö 769. Pp. 109-224. Julkaisu on saatavana myös Internetistä: www.ymparisto.fi/julkaisut ISBN 952-11-1996-9 (nid.) ISBN 952-11-1997-7 (PDF) ISSN 1238-7312 Kannen kuvat / Cover pictures Vasen ylä / Top left: Paljakkaa. Utsjoki. Treeless alpine tundra zone. Utsjoki. Kuva / Photo: Esteri Ohenoja Vasen ala / Down left: Jalopuulehtoa. Parainen, Lenholm. Quercus robur forest. Parainen, Lenholm. Kuva / Photo: Tuomo Niemelä Oikea ylä / Top right: Lehtolohisieni (Laccaria amethystina). Amethyst Deceiver (Laccaria amethystina). Kuva / Photo: Pertti Salo Oikea ala / Down right: Vanhaa metsää. Sodankylä, Luosto. Old virgin forest. Sodankylä, Luosto. Kuva / Photo: Tuomo Niemelä Takakansi / Back cover: Ukonsieni (Macrolepiota procera). -
Pembrokeshire Fungus Recorder Issue 3/2015
Pembrokeshire Fungus Recorder Issue 3/2015 Published quarterly by the Pembrokeshire Fungus Recording Network www.pembsfungi.org.uk Contents 2: Fungus records 5. NBGW field mycology day 7. Study of endophyte fungi in beech (AR) 8. Conservation news Scanning electron microscope image of campion smut © David Spears Introduction After a dry April, rainfall for the quarter 2014/2015 rainfall (mm.) came out close to the 20-year average. 350 Average This edition of our newsletter brings a 300 account of a very successful gathering 2014 2015 of enthusiasts hosted by the National 250 Botanic Garden of Wales. Following 200 plenty of positive feedback from the day, we are now putting together ideas 150 for a similar event in 2016. 100 Anna Rawlings from Swansea Uni- versity has very kindly written an arti- 50 cle on her research into fungi 0 associated with dead beech wood. An- Jan Feb Mar Apr May Jun July Aug Sep Oct Nov Dec na recently collected samples from Orielton Field Centre and has promised (Weather data courtesy of FSC, Orielton) to keep us up to date on progress with her research. As always, our members have come up with some fascinating finds - thanks for these - please keep them coming. Finally, a word of explanation on the image of campion smut (Microbotryum lychnidis- dioicae) spores above. Mike Crutchley sent some samples to a colleague, David Spears, who has an electron microscope at home in Somerset (like you do!). This remarkable image shows the spores which measure just 6-10 microns across. Shame David doesn’t live a bit closer! David Harries, PFRN coordinator ([email protected]) July 2015 Fungus records (Thimble morel) Adam Pollard reported this nice find from woodland in April. -
(Hebden Bridge, West Yorkshire) Using Nextgen DNA Sequencing of Soil Samples
Natural England Commissioned Report NECR258 An assessment of the fungal conservation value of Hardcastle Crags (Hebden Bridge, West Yorkshire) using NextGen DNA sequencing of soil samples First published February 2019 Month XXXX www.gov.uk/natural-england Foreword Natural England commission a range of reports from external contractors to provide evidence and advice to assist us in delivering our duties. The views in this report are those of the authors and do not necessarily represent those of Natural England. Background DNA based applications have the potential to significantly change how we monitor and assess This report should be cited as: biodiversity. These techniques may provide cheaper alternatives to existing species GRIFFITHS, G.W., CAVALLI, O. & monitoring or an ability to detect species that we DETHERIDGE, A.P. 2019. An assessment of the cannot currently detect reliably. fungal conservation value of Hardcastle Crags (Hebden Bridge, West Yorkshire) using NextGen Natural England has been supporting the DNA sequencing of soil samples. Natural development of DNA techniques for a number of England Commissioned Reports, Number 258. years and has funded exploratory projects looking at different taxonomic groups in a range of different ecosystems and habitats. This report presents the results of a survey of fungi of conservation importance at Hardcastle . Crags, West Yorkshire, using DNA based methods. These results are compared to fruiting body surveys which had been carried out in the preceding two years. Natural England Project Manager – Andy Nisbet, Principal Adviser and Evidence Programme Manager, Natural England [email protected] Contractor – Gareth Griffith, Aberystwyth University [email protected] Keywords – DNA, eDNA, sequencing, metabarcoding, monitoring, fungi, waxcaps, grassland Further information This report can be downloaded from the Natural England Access to Evidence Catalogue: http://publications.naturalengland.org.uk/ . -
Waxcaps and Grassland Fungi
5. GREEN WAXCAPS 6. W HITE WAXCAPS 8. BLUE-GREY MUSHROOMS 9. CORAL FUNGI Management for grassland fungi Plantlife Cymru Habitats rich in grassland fungi, especially old grasslands with low Hygrocybe psittacina Parrot Waxcap Hygrocybe virginea Snowy Waxcap Entoloma bloxamii Big Blue Pinkgill S42 Clavaria zollingeri Violet Coral S42 fertility, are susceptible to change, for example through the application of Plantlife Cymru is speaking up for Wales’s fertilisers, ploughing, cessation of grazing, scrub encroachment, tree wild flowers and plants. From the open planting and development. The following guidelines are recommended spaces of our nature reserves to the corridors of the Welsh Assembly, we’re On agricultural grasslands: here to raise their profile, celebrate their beauty and protect their future. Avoid the use of fertilisers, manures and herbicides as these are detrimental to grassland fungi. Wild flowers and plants play a fundamental role for wildlife and their Ensure existing drainage is not impaired as many species require free-draining conditions. colour and character light up our landscapes. But without our help this Retain permanent grassland as cultivation of the soil disrupts or priceless natural heritage is in danger of destroys the underground networks of the fungi – the “mycelia”. being lost. Join us in enjoying the very Grassland fungi can take decades to recover from this. best that nature has to offer. Avoid activities that cause soil compaction which may affect the soil structure and damage the mycelia. Britain’s countryside. Cap: Usually predominantly green turning yellow-green or Cap: White, moist, and initially domed, becoming flatter with age. Cap: A distinctive blue-grey colour when fresh. -
North Kerry Waxcap Survey 2012
Survey of the Grassland Fungi of North Kerry David Mitchel October – November 2012 This project has received support from the Heritage Council under the 2012 Heritage Research Grants Scheme Grant Reference No. R03059 Hygrocybe reidii – a Halloween mushroom? Hygrocybe calyptriformis Hygrocybe ceracea 2 Contents Contents ............................................................................................................................... 3 Background........................................................................................................................... 4 Assessing site quality from fungal data ............................................................................. 5 Aims of this project................................................................................................................ 6 The Study Area..................................................................................................................... 7 History of mycological recording in County Kerry .................................................................. 8 Digitisation of published records ........................................................................................... 9 Cleaning of the Irish Records in the FRDBI......................................................................... 11 Methodology ....................................................................................................................... 12 Results...............................................................................................................................