A Framework for the Examination of Theories Of
Total Page:16
File Type:pdf, Size:1020Kb
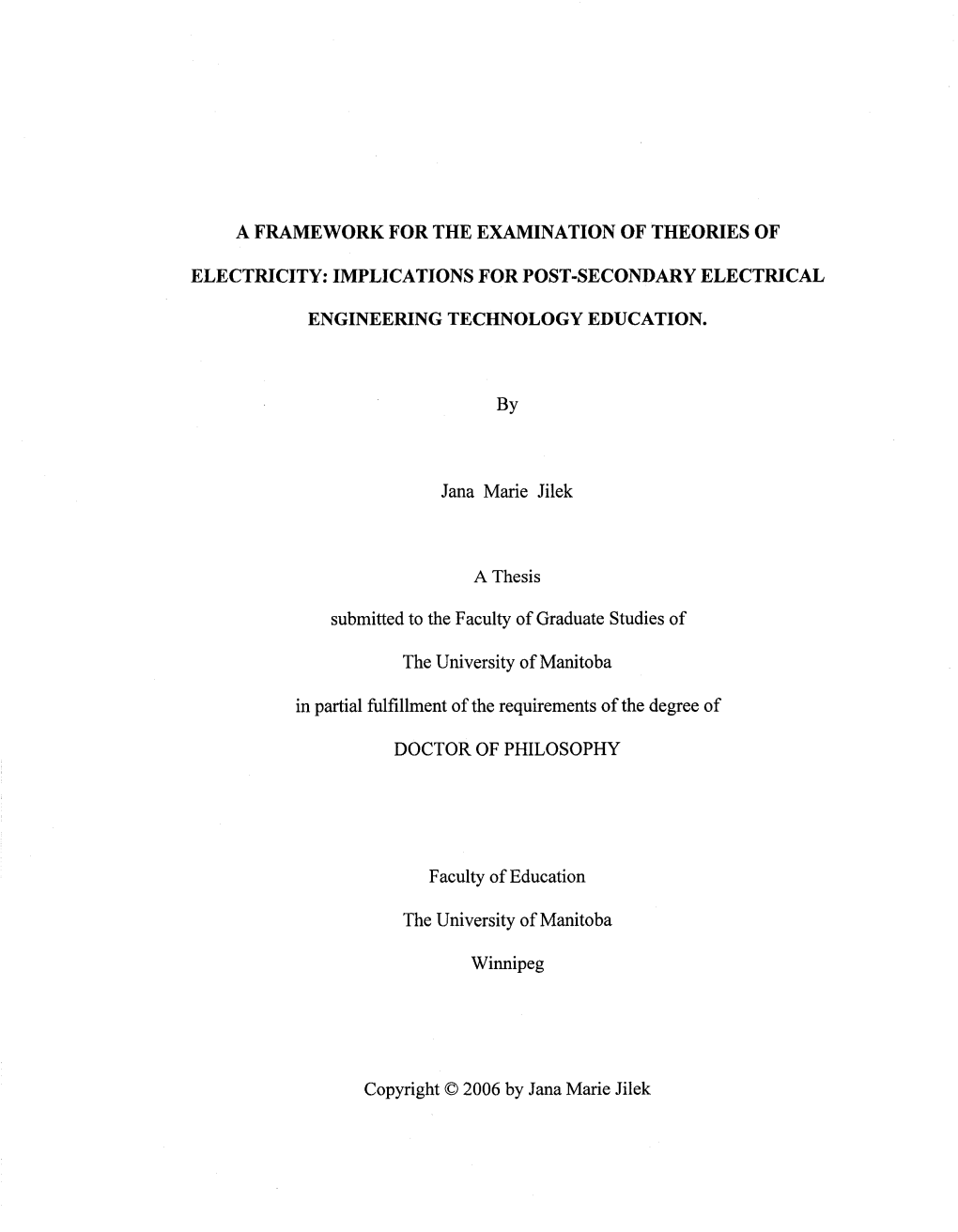
Load more
Recommended publications
-
Keeping the Promise: Phys Rev Completes Online Archive the Physical Review Be Explored
August/September 2001 NEWS Volume 10, No. 8 A Publication of The American Physical Society http://www.aps.org/apsnews Keeping the Promise: Phys Rev Completes Online Archive The Physical Review be explored. The earliest volumes institutions and others to link to Online Archive or of the journals can be examined at APS publications, both current ma- PRL Gets a PROLA is now com- length, in detail and at ease. Histo- terial and PROLA. Authors are also plete: every paper in rians and biographers can track the free to mount their Physical Review New Face every journal that APS expansion of the knowledge of papers on their own sites. has published since physics that took place over the PROLA is composed of scanned 1893 (excepting the previous century in Physical Review. images of the printed journals, op- present and past three Research published in Physical Re- tical character recognition (OCR) years, which are held view by any particular author or material, and a searchable separately for current group or institution can be col- richly-tagged XML bibliographic subscribers) mounted lected and perused with a search database. Each year, another year online in a friendly, of PROLA and a second search of of this material is added to PROLA Bob Kelly/APS powerful, fully search- PROLA team at APS Editorial Office in Ridge, NY: Louise current content. Journalists can ac- from the current subscription con- able system. The project Bogan; Paul Dlug; Mark Doyle, Project Manager; Maxim cess physics Nobel Prize winning tent; 1997 was added in January took just under ten Gregoriev; Gerard Young; Rosemary Clark. -
Analogies in Physics Analysis of an Unplanned Epistemic Strategy
. Analogies in Physics Analysis of an Unplanned Epistemic Strategy Von der Philosophischen Fakultät der Gottfried Wilhelm Leibniz Universität Hannover zur Erlangung des Grades Doktors der Philosophie Dr. phil. Genehmigte Dissertation von Ing. grad. MA Gunnar Kreisel Erscheinungs- bzw. Druckjahr 2021 Referent: Prof. Dr. Mathias Frisch Korreferent: Prof. Dr. Torsten Wilholt Tag der Promotion: 26.10.2020 2 To my early died sister Uta 3 Acknowledgements I could quote only very few by name who have contributed to my work on this thesis, for discussing some of the developed ideas with me or comments on parts of my manuscript. These are in the first place my advisor Mathias Frisch and further Torsten Wilholt, who read critically individual chapters. Much more have contributed by some remarks or ideas mentioned in passing which I cannot assign to someone explicitly and therefore must be left unnamed. Also, other people not named here have supported my work in the one or other way. I think they know who were meant if they read this. A lot of thanks are due to Zoe Vercelli from the International Writing centre at Leibniz University Hannover improving my English at nearly the whole manuscript (some parts are leaved to me because of organisational changes at the writing centre). So, where the English is less correct Zoe could not have had a look on it. Of course, all errors and imprecisions remain in solely my responsibility. 4 Abstract This thesis investigates what tools are appropriate for answering the question how it is possible to develop such a complex theory in physics as the standard model of particle physics with only an access via electromagnetic interaction of otherwise unobservable objects and their interactions it was investigated what the tools are to do this. -
Electrical Machines Lab Ii
Electrical & Electronics Engineering Department BRCM COLLEGE OF ENGINEERING & TECHNOLOGY BAHAL – 127028 ( Distt. Bhiwani ) Haryana, India ELECTRICAL MACHINES LAB II EE-327-F ELECTRICAL MACHINES-II LAB L T P CLASS WORK : 25 Marks 0 0 2 EXAM : 25 Marks TOTAL : 50 Marks DURATION OF EXAM : 3 HRS List of Experiments: 1. Study of the No Load and Block Rotor Test in a Three Phase Slip Ring Induction Motor & draw its circle diagram 2. To Study the Starting of Slip Ring Induction Motor by Rotor Resistance Starter. 3. To Study and Measure Direct and Quadrature Axis Reactance of a 3 phase alternator by Slip Test 4. To Study and Measure Positive, Negative and Zero Sequence Impedance of a Alternator 5. To Study and Measure Synchronous Impedance and Short circuit ratio of Synchronous Generator. 6. Study of Power (Load) sharing between two Three Phase alternators in parallel operation condition 7. Synchronization of two Three Phase Alternators, by a) Synchroscope Method b) Three dark lamp Method c) Two bright one dark lamp Method 8. To plot V- Curve of synchronous motor. 7. To study and verify Load characteristics of Long Shunt & short shunt Commutatively Compound Generator using 3 phase induction motor as prime mover. 10. To perform O.C. test on synchronous generator. And determine the full load regulation of a three phase synchronous generator by synchronous impedance method NOTE: At least 10 experiments are to be performed, with at least 7 from above list, remaining three may either be performed from above list or designed & set by concerned institution as per scope of syllabus. -
Modeling, Analogue and Tests of an Electric Machine
MODELING, ANALOGUE AND TESTS OF AN ELECTRIC MACHINE VOLTAGE CONTROL SYSTEM 'by GRAHAM E.' DAWSON ( j B.A.Sc, University of British. Columbia, 1963. A THESIS SUBMITTED IN PARTIAL FULFILMENT OF THE REQUIREMENTS, FOR THE DEGREE OF MASTER OF APPLIED SCIENCE. in the Department of Electrical Engineering We accept this thesis as conforming to the required standard Members of the Committee Head of the Department .»»«... Members of the Department of Electrical Engineering THE UNIVERSITY OF BRITISH COLUMBIA September, 1966 In presenting this thesis in partial fulfilment of the requirements for an advanced degree at the University of British Columbia, I agree that the Library shall make it freely.avai1able for reference and study. I further agree that permission for ex• tensive copying of this thesis for scholarly purposes may be granted by the Head of my Department or by his representatives.. It is understood that copying or publication of this thesis for finan• cial gain shall not be allowed without my.written permission. Department of Electrical Engineering The University of British Columbia Vancou ve r.,8, Canada Date 7. MM ABSTRACT This thesis is concerned with the modeling, analogue and tests of an interconnected four electric machine voltage control system. Many analogue studies of electric machines have been done but most are concerned with the development of analogue techniques and only a few give substantiation of the validity of the analogue models through comparison of results from analogue studies and from real machine tests. Chapter 2 describes the procedure and the system under study. Chapter 3 describes the methods used for the determination of the electrical and mechanical system parameters. -
FOR PREDICTING INIJJ'c'l'ion MOTOR CHARACTERISTICS I
AN IMPROVED METHOD FOR PREDICTING INIJJ'C'l'ION MOTOR CHARACTERISTICS i AN D ROVED FOR REDICTlNG llTDUC'l'ION MOTO CHA.RAOTERIBT'IOS I' Bachelor of Science :Montana State College BOZtX!lM , , onto.na 1944 j;;)u.bmitt to tho De artnont of leotricnl EngiMeri g Cklaho J..gricultur and rcohenieul College In purtiul Ful.:f'illmen'l; of t e Re0ui ents tor the gree of' STER OF ..:>C ~en 1947 ii OKY.l~fl:\H .1:J:?RO D BY: !GIUCrL TFP..U i M' nwrn· 11, c·m 1, L J B R ,\ • v . DE'C 8 1947 llea! of £fie bepartiii.ent ') r ·> P , .... I ) ~ ~1 J . iii PREFiVJE The induction motor is one o.. f' the most u.se:tul · electrie maohines in industry today. Since its inverrtion in 1888 by !'Jikola Telsa, it has eonstantl;r bee:n replacing other types of machines, both eleotrio .ru1.d meehanieal, as a raeans of supply ing 111sehanioal po;:er. Ma.de in its diversified for.ID1J,. an induction riaotor et:.m be made t;.o fit a.lm.ost any torq_ue-speed requirement. In its most common form., the normal-starting-current. normal-starting-torque, squirrel-cage induction motor., it offers such a.dvantae;es as high effioiency:t practioally constant speed, extremely sirn.plo ope:rationt a11d s:m.s.11 electrical na.intenance requirements. Because e:f the induction moto~•s wide use, it is advantageous to both the roanu:faoturer and the consumer to be able to ascertain, as aoeurately and si:Ll1ply as possible, hmv a given motor vrill operate under varying eondi tions of load. -
Course Description Bachelor of Technology (Electrical Engineering)
COURSE DESCRIPTION BACHELOR OF TECHNOLOGY (ELECTRICAL ENGINEERING) COLLEGE OF TECHNOLOGY AND ENGINEERING MAHARANA PRATAP UNIVERSITY OF AGRICULTURE AND TECHNOLOGY UDAIPUR (RAJASTHAN) SECOND YEAR (SEMESTER-I) BS 211 (All Branches) MATHEMATICS – III Cr. Hrs. 3 (3 + 0) L T P Credit 3 0 0 Hours 3 0 0 COURSE OUTCOME - CO1: Understand the need of numerical method for solving mathematical equations of various engineering problems., CO2: Provide interpolation techniques which are useful in analyzing the data that is in the form of unknown functionCO3: Discuss numerical integration and differentiation and solving problems which cannot be solved by conventional methods.CO4: Discuss the need of Laplace transform to convert systems from time to frequency domains and to understand application and working of Laplace transformations. UNIT-I Interpolation: Finite differences, various difference operators and theirrelationships, factorial notation. Interpolation with equal intervals;Newton’s forward and backward interpolation formulae, Lagrange’sinterpolation formula for unequal intervals. UNIT-II Gauss forward and backward interpolation formulae, Stirling’s andBessel’s central difference interpolation formulae. Numerical Differentiation: Numerical differentiation based on Newton’sforward and backward, Gauss forward and backward interpolation formulae. UNIT-III Numerical Integration: Numerical integration by Trapezoidal, Simpson’s rule. Numerical Solutions of Ordinary Differential Equations: Picard’s method,Taylor’s series method, Euler’s method, modified -
Guides to the Royal Institution of Great Britain: 1 HISTORY
Guides to the Royal Institution of Great Britain: 1 HISTORY Theo James presenting a bouquet to HM The Queen on the occasion of her bicentenary visit, 7 December 1999. by Frank A.J.L. James The Director, Susan Greenfield, looks on Front page: Façade of the Royal Institution added in 1837. Watercolour by T.H. Shepherd or more than two hundred years the Royal Institution of Great The Royal Institution was founded at a meeting on 7 March 1799 at FBritain has been at the centre of scientific research and the the Soho Square house of the President of the Royal Society, Joseph popularisation of science in this country. Within its walls some of the Banks (1743-1820). A list of fifty-eight names was read of gentlemen major scientific discoveries of the last two centuries have been made. who had agreed to contribute fifty guineas each to be a Proprietor of Chemists and physicists - such as Humphry Davy, Michael Faraday, a new John Tyndall, James Dewar, Lord Rayleigh, William Henry Bragg, INSTITUTION FOR DIFFUSING THE KNOWLEDGE, AND FACILITATING Henry Dale, Eric Rideal, William Lawrence Bragg and George Porter THE GENERAL INTRODUCTION, OF USEFUL MECHANICAL - carried out much of their major research here. The technological INVENTIONS AND IMPROVEMENTS; AND FOR TEACHING, BY COURSES applications of some of this research has transformed the way we OF PHILOSOPHICAL LECTURES AND EXPERIMENTS, THE APPLICATION live. Furthermore, most of these scientists were first rate OF SCIENCE TO THE COMMON PURPOSES OF LIFE. communicators who were able to inspire their audiences with an appreciation of science. -
TRUET Bo THOMPSON \'• Bachelor of Science Louisiana Poiytechnic Institute 1948
i SOME ASPECTS OF SINGLE PH.A.SE INDUCTION MOTOR THEORY By TRUET Bo THOMPSON \'• Bachelor of Science Louisiana Poiytechnic Institute 1948. Submitted to ·the Faculty of the Graduate School of the Oklahoma Agricultural and Mechanical College in Partial Fulfillment of the Requirements for the Degree of MASTER OF SCIENCE 1950 ii SOME ASPECTS OF SINGLE PHASE INDUCTION MOTOR THEORY ' TRUET Bo THOMPSON MASTER OF SCIENCE 1950 THESIS AND ABSTRACT APPROVED: FacultYi~~ De~f!ue Graduate School 266841 iii PREFACE For more than 50 years the two distinct theories of single phase motor operation, the cross-field theory and the double revolving field theory, have been used to explain the charac teristics of these motors. Neither is entirely satisfactory either in its calculation of characteristics or in the physical conception of the motor's operation. The complexity and actual mystery involved have defied through this half century efforts to si~lify and correlate completely the theories now extant. This paper is designed, not to perform this task, nor to develop a new concept, but rather to bring together a· few ideas which have been helpful in the work which was carried on in the belief that there can be a more satisfactory explanation of single phase moto~ operation. iv ACKNOWLEDGMENT The writer wishes to express his sincere appreciation to Professor C& Fo Cameron for his encouragement, his reading of this material and his many helpful suggestions concerning it., V TABLE OF CONTENTS CHA PTER I. • • • • • • • . • . 1 The Double Revolving Field. • . • ••••. 1 Two Oppositely Rotating Fields in a Single Stator. • • 13 Two Oppositely Rotating Fields in Two Stators • • • • • 25 Mathematical Development by Equivalent Circuit •• • • • 29 Another Approach to Performance Equations . -
November 2019
A selection of some recent arrivals November 2019 Rare and important books & manuscripts in science and medicine, by Christian Westergaard. Flæsketorvet 68 – 1711 København V – Denmark Cell: (+45)27628014 www.sophiararebooks.com AMPÈRE, André-Marie. THE FOUNDATION OF ELECTRO- DYNAMICS, INSCRIBED BY AMPÈRE AMPÈRE, Andre-Marie. Mémoires sur l’action mutuelle de deux courans électri- ques, sur celle qui existe entre un courant électrique et un aimant ou le globe terres- tre, et celle de deux aimans l’un sur l’autre. [Paris: Feugeray, 1821]. $22,500 8vo (219 x 133mm), pp. [3], 4-112 with five folding engraved plates (a few faint scattered spots). Original pink wrappers, uncut (lacking backstrip, one cord partly broken with a few leaves just holding, slightly darkened, chip to corner of upper cov- er); modern cloth box. An untouched copy in its original state. First edition, probable first issue, extremely rare and inscribed by Ampère, of this continually evolving collection of important memoirs on electrodynamics by Ampère and others. “Ampère had originally intended the collection to contain all the articles published on his theory of electrodynamics since 1820, but as he pre- pared copy new articles on the subject continued to appear, so that the fascicles, which apparently began publication in 1821, were in a constant state of revision, with at least five versions of the collection appearing between 1821 and 1823 un- der different titles” (Norman). The collection begins with ‘Mémoires sur l’action mutuelle de deux courans électriques’, Ampère’s “first great memoir on electrody- namics” (DSB), representing his first response to the demonstration on 21 April 1820 by the Danish physicist Hans Christian Oersted (1777-1851) that electric currents create magnetic fields; this had been reported by François Arago (1786- 1853) to an astonished Académie des Sciences on 4 September. -
EE6402 – TRANSMISSION and DISTRIBUTION UNIT-I 1. Why High Voltage Is Preferred for Power Transmission? (MJ15, ND15, MJ16) 2. S
EE6402 – TRANSMISSION AND DISTRIBUTION UNIT-I 1. Why high voltage is preferred for power transmission? (MJ15, ND15, MJ16) As voltage increases, current flow through the line decreases and I2R loss reduces. So transmission efficiency increases. Therefore high voltage is preferred for power transmission. 2. State the disadvantages of HVDC transmission. (ND10) i) High cost of terminal equipment ii) Converters require considerable reactive power. iii) Harmonics are generated which requires filters. iv) Converters do not have overload capacity. 3. Why the transmission lines 3 phase 3 wire circuits while distribution lines are 3ϕ, 4 wire circuits? (ND10, ND13) A Balanced 3 phase circuit does not require the neutral conductor, as the instantaneous sum of the 3 line currents are zero. Therefore the transmission lines and feeders are 3 phase 3 wire circuits. The distributors are 3 phase 4 wire circuits because a neutral wire is necessary to supply the 1 phase loads of domestic and commercial consumers. 4. Define the terms feeders and service mains. (ND11, ND15) Feeders: It is a conductor which connects the substation to the area where power is to be distributed. Generally, no tappings are taken from the feeder. Service mains: A service main is generally a small cable which connects the distributor to the consumer’s terminal. 5. List out the advantages of high voltage A.C transmission. (ND11) i) The power can be generated at high voltages. ii) The maintenance of ac substation is easy and cheaper. 6. What is ring main distributor? (ND12, MJ17) In ring main distributor, the distributor is in the form of a closed ring. -
Historical Perspective of Electricity
B - Circuit Lab rev.1.04 - December 19 SO Practice - 12-19-2020 Just remember, this test is supposed to be hard because everyone taking this test is really smart. Historical Perspective of Electricity 1. (1.00 pts) The first evidence of electricity in recorded human history was… A) in 1752 when Ben Franklin flew his kite in a lightning storm. B) in 1600 when William Gilbert published his book on magnetism. C) in 1708 when Charles-Augustin de Coulomb held a lecture stating that two bodies electrified of the same kind of Electricity exert force on each other. D) in 1799 when Alessandro Volta invented the voltaic pile which proved that electricity could be generated chemically. E) in 1776 when André-Marie Ampère invented the electric telegraph. F) about 2500 years ago when Thales of Miletus noticed that a piece of amber attracted straw or feathers when he rubbed it with cloth. 2. (3.00 pts) The word electric… (Mark ALL correct answers) A) was first used in printed text when it was published in William Gilber’s book on magnetism. B) comes from the Greek word ήλεκτρο (aka “electron”) meaning amber. C) adapted the meaning “charged with electricity” in the 1670s. D) was first used by Nicholas Callen in 1799 to describe mail transmitted over telegraph wires, “electric-mail” or “email”. E) was cast in stone by Greek emperor Julius Caesar when he knighted Archimedes for inventing the electric turning lathe. F) was first used by Michael Faraday when he described electromagnetic induction in 1791. 3. (5.00 pts) Which five people, who made scientific discoveries related to electricity, were alive at the same time? (Mark ALL correct answers) A) Charles-Augustin de Coulomb B) Alessandro Volta C) André-Marie Ampère D) Georg Simon Ohm E) Michael Faraday F) Gustav Robert Kirchhoff 4. -
B. Tech Electrical.Pdf
JECRC University Course Structure for Electrical Engineering (B.Tech.) JECRC UNIVERSITY Faculty of Engineering & Technology B.Tech in Electrical Engineering Teaching Scheme Semester III Subject Code Subject Contact Hrs Credits L-T-P Electronics Devices & Circuits 3-1-2 5 Circuit Analysis – I 3-1-0 4 Electrical Machines – I 3-1-2 5 Electrical Measurements 3-1-2 5 Mathematics – III 3-1-0 4 Computer Programming – I 3-0-2 4 Total 18-5-8 27 JECRC UNIVERSITY Faculty of Engineering & Technology B.Tech in Electrical Engineering Teaching Scheme Semester IV Subject Code Subject Contact Hrs Credits L-T-P Analogue Electronics 3-1-2 5 Digital Electronics 3-0-2 4 Circuit Analysis – II 3-1-0 4 Electrical Machines – II 3-1-2 5 Advanced Mathematics 3-1-0 4 Generation of Electric Power 3-0-0 3 Total 18-4-6 25 JECRC UNIVERSITY Faculty of Engineering & Technology B.Tech in Electrical Engineering Teaching Scheme Semester V Subject Code Subject Contact Hrs Credits L-T-P Power Electronics-I 3-1-2 5 Microprocessor & Computer 3-0-2 4 Architecture Transmission & Distribution – I 3-1-0 4 Control Systems 3-1-2 5 Utilization of Electrical Power 3-0-0 3 Digital Signal Processing 3-0-0 3 Total 18-3-6 24 JECRC UNIVERSITY Faculty of Engineering & Technology B.Tech in Electrical Engineering Teaching Scheme Semester VI Subject Code Subject Contact Hrs Credits L-T-P Power Electronics –II 3-1-2 5 Power System Analysis 3-1-2 5 EHV AC/DC Transmission 3-0-0 3 Switch Gear & protection 3-0-0 3 Instrumentation 3-0-0 3 Transmission & Distribution – II 3-1-0 4 Economics 0-0-2 1