Piper, Munchen, 1977
Total Page:16
File Type:pdf, Size:1020Kb
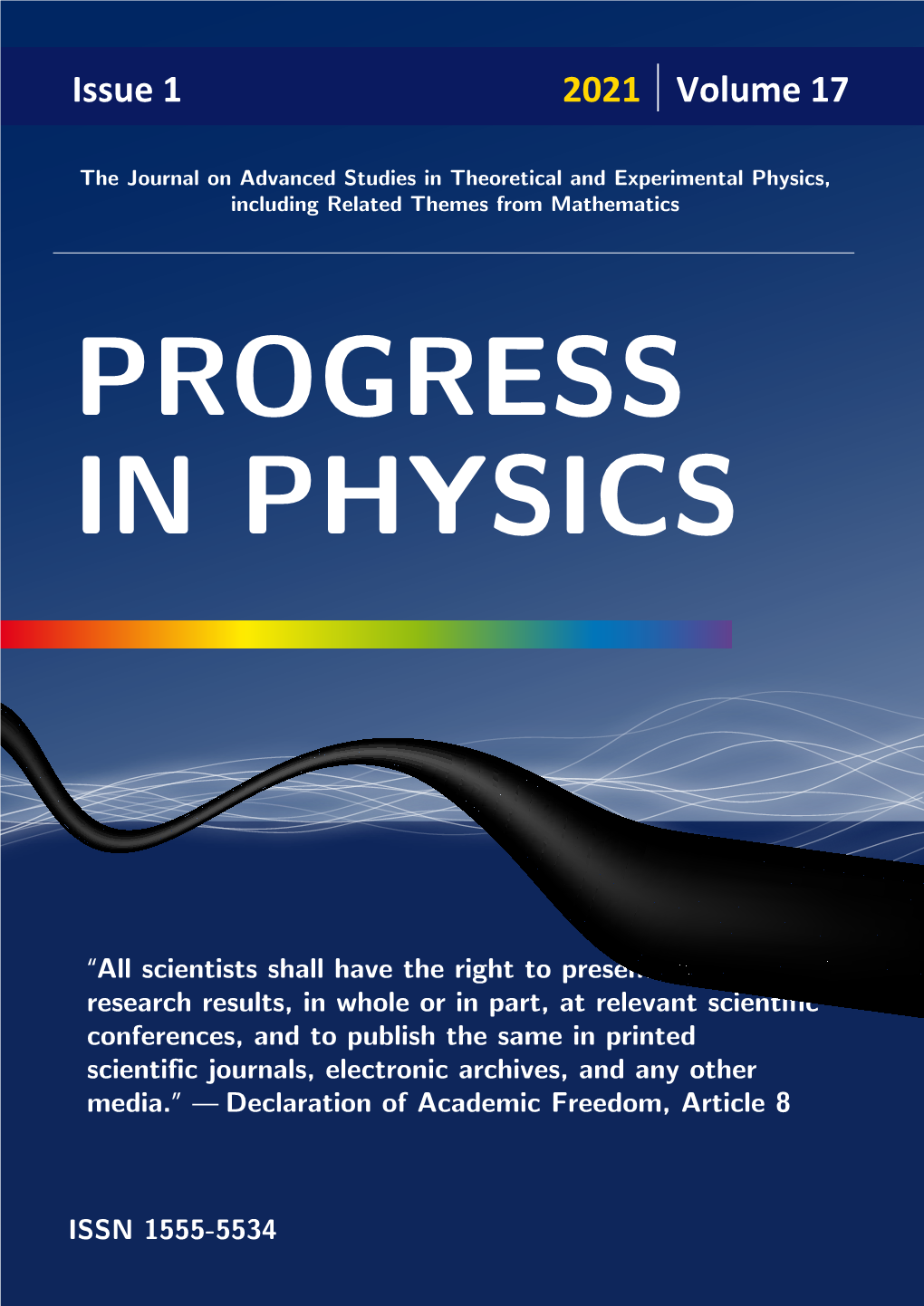
Load more
Recommended publications
-
Physics of Transcendental Numbers Meets Gravitation
Issue 1 (April) PROGRESS IN PHYSICS Volume 17 (2021) Physics of Transcendental Numbers Meets Gravitation Hartmut Muller¨ E-mail: [email protected] Transcendental ratios of physical quantities can provide stability in complex dynamic systems because they inhibit the occurrence of destabilizing resonance. This approach leads to a fractal scalar field that affects any type of physical interaction and allows re- formulating and resolving some unsolved tasks in celestial mechanics and astrophysics. We verify the model claims on the gravitational constants and the periods of orbital and rotational motion of the planets, planetoids and large moons of the solar system as well as the orbital periods of exoplanets and the gravitational constants of their stars. Introduction many pairs of orbital periods and distances that fulfill Ke- pler’s laws. Einstein’s field equations do not reduce the theo- Despite the abundance of theoretical approaches engaged to retical variety of possible orbits, but increases it even more. explain the origin of gravitational interaction dealing with But now, after the discovery of thousands of exoplanetary superstrings, chameleons or entropic forces [1], the commu- systems, we can recognize that the current distribution of the nity of physicists still expects compatibility for centuries: any planetary and lunar orbits in our solar system is not acciden- modern theory must allow deriving Newton’s law of univer- tal. Many planets in the extrasolar systems like Trappist 1 or sal gravitation as classic approximation. In the normal case Kepler 20 have nearly the same orbital periods as the large of weak gravity and low velocities, also Einstein’s field equa- moons of Jupiter, Saturn, Uranus and Neptune [4]. -
The Official Publication of the Hamilton Centre, Royal Astronomical Society of Canada Volume 47, Issue 8: June, 2015
Orbit The Official Publication of The Hamilton Centre, Royal Astronomical Society of Canada Volume 47, Issue 8: June, 2015 Issue Number 8, June, 2015 Roger Hill, Editor Here we go...AstroCATS 2015! If you haven’t been to one before, then here’s what you should know: AstroCATS is a great place to check out equip- ment. It’s a great place to hear some great talks by some great speakers; do some solar observing; meet with some old friends and make some new ones. Unfortunately for me, it’s increasingly looking like I will indeed have to work that weekend. I may be able to make the final couple of hours, so I’m hoping there are still some bargains left. If, unlike me, you have some time to attend AstroCATS this year, the organizers sure could use your help. Go to http://www.astrocats.ca/volunteer.html and let them know your availability. It’s always fun, and while it may be my imagination, I think volunteers may just get a little extra discount from the vendors. Of course, with the venue being the Ontario Science Centre, there’s always lots more to go for than just astronomy! Head on over to the website—www.astrocats.ca—and check everything out. One final thing...NEAF, in the US, is renowned for vendors using it to announce new products. I’m hearing that a Cana- dian company is going to use AstroCATS to do the same thing...and not just one product, but 3! I had the opportunity after the May long weekend to do some driving into the US with my son. -
September 2017 BRAS Newsletter
September 2017 Issue September 2017 Next Meeting: Monday, September 11th at 7PM at HRPO nd (2 Mondays, Highland Road Park Observatory) September Program: GAE (Great American. Eclipse) Membership Reports. Club members are invited to “approach the mike. ” and share their experiences travelling hither and thither to observe the August total eclipse. What's In This Issue? HRPO’s Great American Eclipse Event Summary (Page 2) President’s Message Secretary's Summary Outreach Report - FAE Light Pollution Committee Report Recent Forum Entries 20/20 Vision Campaign Messages from the HRPO Spooky Spectrum Observe The Moon Night Observing Notes – Draco The Dragon, & Mythology Like this newsletter? See past issues back to 2009 at http://brastro.org/newsletters.html Newsletter of the Baton Rouge Astronomical Society September 2017 The Great American Eclipse is now a fond memory for our Baton Rouge community. No ornery clouds or“washout”; virtually the entire three-hour duration had an unobstructed view of the Sun. Over an hour before the start of the event, we sold 196 solar viewers in thirty-five minutes. Several families and children used cereal box viewers; many, many people were here for the first time. We utilized the Coronado Solar Max II solar telescope and several nighttime telescopes, each outfitted with either a standard eyepiece or a “sun funnel”—a modified oil funnel that projects light sent through the scope tube to fabric stretched across the front of the funnel. We provided live feeds on the main floor from NASA and then, ABC News. The official count at 1089 patrons makes this the best- attended event in HRPO’s twenty years save for the historic Mars Opposition of 2003. -
Project Icarus: Astronomical Considerations Relating to the Choice of Target Star
Project Icarus: Astronomical Considerations Relating to the Choice of Target Star I. A. Crawford Department of Earth and Planetary Sciences, Birkbeck College London, Malet Street, London, WC1E 7HX Abstract In this paper we outline the considerations required in order to select a target star system for the Icarus interstellar mission. It is considered that the maximum likely range for the Icarus vehicle will be 15 light‐years, and a list is provided of all known stars within this distance range. As the scientific objectives of Icarus are weighted towards planetary science and astrobiology, a final choice of target star(s) cannot be made until we have a clearer understanding of the prevalence of planetary systems within 15 light‐ years of the Sun, and we summarize what is currently known regarding planetary systems within this volume. We stress that by the time an interstellar mission such as Icarus is actually undertaken, astronomical observations from the solar system will have provided this information. Finally, given the high proportion of multiple star systems within 15 light‐years (including the closest of all stars to the Sun in the α Centauri system), we stress that a flexible mission architecture, able to visit stars and accompanying planets within multiple systems, is desirable. This paper is a submission of the Project Icarus Study Group. Keywords: Interstellar travel; nearby stars; extrasolar planets; astrobiology 1. Introduction The Icarus study is tasked with designing an interstellar space vehicle capable of making in situ scientific investigations of a nearby star and accompanying planetary system [1,2]. This paper outlines the considerations which will feed into the choice of the target star, the choice of which will be constrained by a number of factors. -
The Milky Way the Milky Way's Neighbourhood
The Milky Way What Is The Milky Way Galaxy? The.Milky.Way.is.the.galaxy.we.live.in..It.contains.the.Sun.and.at.least.one.hundred.billion.other.stars..Some.modern. measurements.suggest.there.may.be.up.to.500.billion.stars.in.the.galaxy..The.Milky.Way.also.contains.more.than.a.billion. solar.masses’.worth.of.free-floating.clouds.of.interstellar.gas.sprinkled.with.dust,.and.several.hundred.star.clusters.that. contain.anywhere.from.a.few.hundred.to.a.few.million.stars.each. What Kind Of Galaxy Is The Milky Way? Figuring.out.the.shape.of.the.Milky.Way.is,.for.us,.somewhat.like.a.fish.trying.to.figure.out.the.shape.of.the.ocean.. Based.on.careful.observations.and.calculations,.though,.it.appears.that.the.Milky.Way.is.a.barred.spiral.galaxy,.probably. classified.as.a.SBb.or.SBc.on.the.Hubble.tuning.fork.diagram. Where Is The Milky Way In Our Universe’! The.Milky.Way.sits.on.the.outskirts.of.the.Virgo.supercluster..(The.centre.of.the.Virgo.cluster,.the.largest.concentrated. collection.of.matter.in.the.supercluster,.is.about.50.million.light-years.away.).In.a.larger.sense,.the.Milky.Way.is.at.the. centre.of.the.observable.universe..This.is.of.course.nothing.special,.since,.on.the.largest.size.scales,.every.point.in.space. is.expanding.away.from.every.other.point;.every.object.in.the.cosmos.is.at.the.centre.of.its.own.observable.universe.. Within The Milky Way Galaxy, Where Is Earth Located’? Earth.orbits.the.Sun,.which.is.situated.in.the.Orion.Arm,.one.of.the.Milky.Way’s.66.spiral.arms..(Even.though.the.spiral. -
The Detectability of Nightside City Lights on Exoplanets
Draft version September 6, 2021 Typeset using LATEX twocolumn style in AASTeX63 The Detectability of Nightside City Lights on Exoplanets Thomas G. Beatty1 1Department of Astronomy and Steward Observatory, University of Arizona, Tucson, AZ 85721; [email protected] ABSTRACT Next-generation missions designed to detect biosignatures on exoplanets will also be capable of plac- ing constraints on the presence of technosignatures (evidence for technological life) on these same worlds. Here, I estimate the detectability of nightside city lights on habitable, Earth-like, exoplan- ets around nearby stars using direct-imaging observations from the proposed LUVOIR and HabEx observatories. I use data from the Soumi National Polar-orbiting Partnership satellite to determine the surface flux from city lights at the top of Earth's atmosphere, and the spectra of commercially available high-power lamps to model the spectral energy distribution of the city lights. I consider how the detectability scales with urbanization fraction: from Earth's value of 0.05%, up to the limiting case of an ecumenopolis { or planet-wide city. I then calculate the minimum detectable urbanization fraction using 300 hours of observing time for generic Earth-analogs around stars within 8 pc of the Sun, and for nearby known potentially habitable planets. Though Earth itself would not be detectable by LUVOIR or HabEx, planets around M-dwarfs close to the Sun would show detectable signals from city lights for urbanization levels of 0.4% to 3%, while city lights on planets around nearby Sun-like stars would be detectable at urbanization levels of & 10%. The known planet Proxima b is a particu- larly compelling target for LUVOIR A observations, which would be able to detect city lights twelve times that of Earth in 300 hours, an urbanization level that is expected to occur on Earth around the mid-22nd-century. -
A Green Line Around the Box Means a Habitable Planet Exists in the Starsystem
Hussey 575 BD+30 2494 BD+24 2733 1.5 BD+14 2774 2.5 1.5 1.5 Ross 512 1.5 WO 9502 1.5 BD+17 2785 2 BD+15 3108 G136-101 Sigma Bootis BD+30 2512 1 Struve 1785 1.5 1 2.5 via LP441.33 1.5 BD+24 2786 1.5 1.5 1.5 1.5 1.5 45 Bootis L1344-37 GJ 3966 Ross 863 1.5 Lambda Serpentis Wolf 497 BD+25 2874 1.5 3 via V645 Herc Gliese 625 1 1.5 3.5 via GJ 3910 3 via V645 Herc 1.5 BD-9 3413 GJ-1179 Zeta Herculis 1.5 Eta Bootes 1 Rho Coronae Borealis 1.5 1.5 2.5 via Giclas 258.33 Luyten 758-105 BD+54 1716 1 BD+25 3173 Giclas 179-43 1.5 1.5 1.5 1 3 via Ross 948 3 via WO 9564 3 via GJ 3796 3 via Ross 948 Eta Coronae Borealis Chi Draconis 3 via HYG86887 1 3.5 via V645 Herc 1 Gamma Serpentis 2 BD+17 2611 (VESTA) Arcturus 3 via V645 Herc 3 via BD+21 2763 3.5 via BD+0 2989, DT Virginis 4 via HYG86887&8 Struve 2135 BD+33 2777 14 Herculis Porimma (Gamma Virginis) 3.5 via BD+45 2247 3 via BD+41 2695 1.5 3.5 BD+60 1623 BD+53 1719 THETIS 1.5 Chi Hercules 2 3.5 1.5 1.5 BD+38 3095 3.5 via BD+46 1189 Mu Herculis 4 via WO 9564 CR Draconis BD+52 2045 3 via BD+43 2796 1.5 3 via Giclas 179-43 BD+36 2393 Queen Isabel Star Sigma Draconis 3.5 via BD+43 2796 CE Bootes 1.5 BD+19 2881 1 1.5 1.5 1.5 2.5 I Bootis via BD+45 2247 BD+47 2112 1 4 via EGWD 329, CR Draconis 3 via LP 272-25 4.5 via Ross 948, L 910-10 44 Bootis Aitkens Double Star 1 ADS 10288 3 via BD+61 2068 1.5 Gilese 625 1.5 Vega 3 via Kuiper 79 3 via Giclas 179-43 4 via BD+46 1189, BD+50 2030 Ross 52 Theta Draconis 2 EV Lacertae Gliese 892 BD+39 2947 3 via BD+18 3421 1.5 0.5 Theta Bootis 1.5 3 via EV Lacertae 2.5 via -
Scientific American
Medicine Climate Science Electronics How to Find the The Last Great Hacking the Best Treatments Global Warming Power Grid Winner of the 2011 National Magazine Award for General Excellence July 2011 ScientificAmerican.com PhysicsTHE IntellıgenceOF Evolution has packed 100 billion neurons into our three-pound brain. CAN WE GET ANY SMARTER? www.diako.ir© 2011 Scientific American www.diako.ir SCIENTIFIC AMERICAN_FP_ Hashim_23april11.indd 1 4/19/11 4:18 PM ON THE COVER Various lines of research suggest that most conceivable ways of improving brainpower would face fundamental limits similar to those that affect computer chips. Has evolution made us nearly as smart as the laws of physics will allow? Brain photographed by Adam Voorhes at the Department of Psychology, Institute for Neuroscience, University of Texas at Austin. Graphic element by 2FAKE. July 2011 Volume 305, Number 1 46 FEATURES ENGINEERING NEUROSCIENCE 46 Underground Railroad 20 The Limits of Intelligence A peek inside New York City’s subway line of the future. The laws of physics may prevent the human brain from By Anna Kuchment evolving into an ever more powerful thinking machine. BIOLOGY By Douglas Fox 48 Evolution of the Eye ASTROPHYSICS Scientists now have a clear view of how our notoriously complex eye came to be. By Trevor D. Lamb 28 The Periodic Table of the Cosmos CYBERSECURITY A simple diagram, which celebrates its centennial this 54 Hacking the Lights Out year, continues to serve as the most essential conceptual A powerful computer virus has taken out well-guarded tool in stellar astrophysics. By Ken Croswell industrial control systems. -
Scientific and Societal Benefits of Interstellar Exploration
See discussions, stats, and author profiles for this publication at: https://www.researchgate.net/publication/275652484 Scientific and Societal Benefits of Interstellar Exploration Chapter · September 2014 CITATION READS 1 29 1 author: Ian A Crawford Birkbeck, University of Lon… 328 PUBLICATIONS 2,410 CITATIONS SEE PROFILE Available from: Ian A Crawford Retrieved on: 03 June 2016 Beyond the Boundary Chapter1 Scientific and Societal Benefits of Interstellar Exploration Ian A Crawford he growing realisation that planets are common companions of stars [1–2] has reinvigorated astronautical studies of how they might be explored using Tinterstellar space probes (for reviews see references [3-7], and also other chap- ters in this book). The history of Solar System exploration to-date shows us that spacecraft are required for the detailed study of planets, and it seems clear that we will eventually require spacecraft to make in situ studies of other planetary systems as well. The desirability of such direct investigation will become even more apparent if future astronomical observations should reveal spectral evidence for life on an apparently Earth-like planet orbiting a nearby star. Definitive proof of the existence of such life, and studies of its underlying biochemistry, cellular structure, ecological diversity and evolutionary history will require in situ inves- tigations to be made [8]. This will require the transportation of sophisticated scientific instruments across interstellar space. Moreover, in addition to the scientific reasons for engaging in a programme of interstellar exploration, there also exist powerful societal and cultural motivations. Most important will be the stimulus to art, literature and philosophy, and the general enrichment of our world view, which inevitably results from expanding the horizons of human experience [9,10]. -
44 Closest Stars and How They Compare to Our Sun
44 CLOSEST STARS AND HOW THEY COMPARE TO OUR SUN R = Solar radius (a unit of distance to express the size of stars relative to the sun) L = Solar luminosity (a unit of radiant flux used SUN System/constellation to compare the luminosity of stars, galaxies, Solar System Potential planets and other celestial objects in terms of the sunʼs output) 8 Distance From Earth 8.317 light-minutes EARTH 1R (432,288 miles) s) -year (light Proxima Centauri TH EAR Alpha Centauri OM E FR 1 ANC DIST 4.244 light-years 0.001 0.01 0.1 0.2 0.3 0.4 0.5 0.6 0.7 0.8 0.9 10 25 0.1542R 0.00005L L <=0.0001 α Centauri A (Rigil Kentaurus) 1 Alpha Centauri L 4.365 light-years 1.223R 1.519 L α Centauri B (Toliman) 5 Alpha Centauri light-years 4.37 light-years 0.863R 0.5002L Bernard’s Star Ophiuchus 1 5.957 light-years 0.196R 0.0035L Wolf 359 (CN Leonis) Leo 2 7.856 light-years 0.16R 0.0014L Lalande 21185 Ursa Major 1 8.307 light-years 0.393R 0.026L Sirius A Canis Major Luyten 726-8A 8.659 light-years Cetus 1.711 R 25.4L 8.791 light-years 0.14R 0.00004L Sirius B Canis Major 8.659 light-years Luyten 726-8B 0.0084R 0.056L Cetus 8.791 light-years 0.14R 0.00004L Ross 154 Sagittarius 9.7035 light-years 0.24R 0.0038L 10 light-years Epsilon Eridani Eridanus Ross 248 2 Andromeda 10.446 light-years 10.2903 light-years 0.735R 0.34L 0.16R 0.0018L Lacaille 9352 Piscis Austrinus 3 10.7211 light-years Ross 128 0.47R 0.0367L Virgo 1 EZ Aquarii A 11 light-years Aquarius 61 Cygni A 0.1967R 0.00362L 11.1 light-years Cygnus 0.175R 0.000087L 3 (part of triple star system) 11.4 light-years -
ED210145.Pdf
DOCUMENT NESUME ED 210 145 LE 033 913 AUTHOR Mayer, Victor J., Ed. TITLE Activity Sourcebook for\Zarth Science. Science Education Information Report. /NSZITUTION ERIC. Clearinghouse for Science, Mathematics, and Environmental Education, Columbus, Ohio. SPONS AGENCY National Inst. of Education (ED), washirgtor, D.C. PUB DATE Dec BO CONZRACT 400-79-0004 NOTE 249p. AVAILABLE FROM Information. Reference Center (ERIC/IRC) ,The Ohio State Univ., 1200 Chambers Rd 3rd Floor, Columbus, OH 43212 ($7.75). EDPS PPICE MF01/PC10 Plus Postage. DESCRIPTORS Astronomy; Climate; *Earth Science; *Field Studies: Geology: *Meteorology: Oceanography; Physical Geography; *Science Activities; Science Education; Secondary Education: *Secondary School Science: Seismology; Space Sciences IDENT/F/ITS *Plate Tectonics: Space Photography ABSZRACT Designed to provide teachers of earth science with activities and information that will assist them in keeFing their curr4cula up to date, this publication contains activities grouped into six chapters. Chapter titles are:(1) Weather and Climate, (2) Oceans,(3) The Earth and Its Surface, (4) Plate Tectonics, (5) Uses of Space Photography, and (6)Space. Each activity has been set in the same general format (introduction, objectives, materials, procedure, and, for some activities, review or summary questions) . Some activities are new; others have been standard for years but are located in publications ro longer readily available to teachers. (PB) *********************************************************************** Reproductiors -
The Hertzsprung-Russell Diagram
The Hertzsprung-Russell Diagram A. Luminosity, Temperature, and Size Introduction In the early part of this century, two astronomers, one Danish and one American, invented a diagram showing the basic characteristics of stars. The color-magnitude diagram, often called the Hertzsprung-Russell (HR) diagram in their honor, has proved to be the Rosetta stone of stellar astronomy. The purpose of this exercise is to give you some familiarity with the diagram. In addition, you will be asked to investigate the types of biases in measurement used to construct this diagram. Biases are especially important in understanding astronomical data. Unlike laboratory sciences, astronomical experiments must be conducted under the conditions the Universe gives us. Another way to think about this is that astronomy has no test tube! Since the astronomer has no direct control over the experiment, it is imperative that he or she understands the prejudices introduced into the data by the human perspective. However, biases of measurement are found in many other fields of science. An example of a biased study would be to find the weight vs. age relation for all Americans by weighing only members of health clubs. Most active health club members tend to be more fit than the average, and so the average derived would be lower than the true average weight of Americans. This exercise asks you to make comparisons between two different samples of stars. The bright star table was selected on the basis of apparent brightness, NOT the luminosity of the stars. The near star table is all stars within 5 parsecs (about 15-16 light years) from the Sun.