Scanning Probe Microscopy, 1960-2000
Total Page:16
File Type:pdf, Size:1020Kb
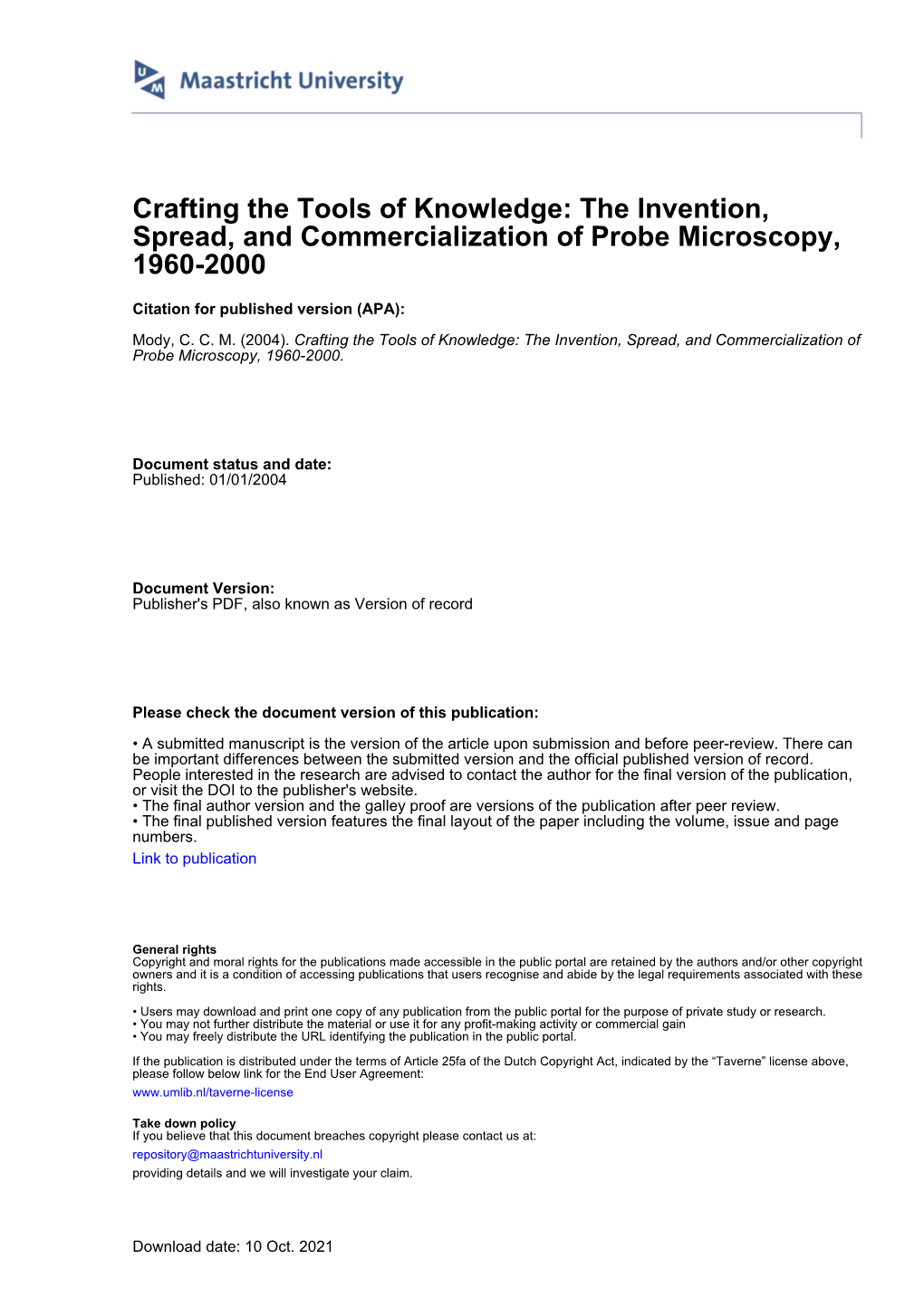
Load more
Recommended publications
-
30 Years of Moving Individual Atoms
FEATURES 30 YEARS OF MOVING INDIVIDUAL ATOMS 1 2 l Christopher Lutz and Leo Gross – DOI: https://doi.org/10.1051/epn/2020205 l 1 IBM Research – Almaden, San Jose, California, USA l 2 IBM Research – Zurich,¨ 8803 Ruschlikon,¨ Switzerland In the thirty years since atoms were first positioned individually, the atom-moving capability of scanning probe microscopes has grown to employ a wide variety of atoms and small molecules, yielding custom nanostructures that show unique electronic, magnetic and chemical properties. his year marks the thirtieth anniversary of the publication by IBM researchers Don Eigler and Erhard Schweizer showing that individ- Tual atoms can be positioned precisely into chosen patterns [1]. Tapping the keyboard of a personal computer for 22 continuous hours, they controlled the movement of a sharp tungsten needle to pull 35 individ- ual xenon atoms into place on a surface to spell the letters “IBM” (Figure 1). Eigler and Schweitzer’s demonstration set in motion the use of a newly invented tool, called the scanning tunneling microscope (STM), as the workhorse for nanoscience research. But this achievement did even more than that: it changed the way we think of atoms. m FIG. 2: The STM that Don Eigler and coworkers used to position atoms. The It led us to view them as building blocks that can be tip is seen touching its reflection in the sample’s surface. (Credit: IBM) arranged the way we choose, no longer being limited by the feeling that atoms are inaccessibly small. with just one electron or atom or (small) molecule. FIG. -
Small Wonders, Endless Frontiers
Small Wonders, Endless Frontiers A Review of the National Nanotechnology Initiative Committee for the Review of the National Nanotechnology Initiative Division on Engineering and Physical Sciences National Research Council NATIONAL ACADEMY PRESS Washington, D.C. NOTICE: The project that is the subject of this report was approved by the Governing Board of the National Research Council, whose members are drawn from the councils of the National Academy of Sciences, the National Academy of Engineering, and the Institute of Medicine. The members of the committee responsible for the report were chosen for their special competences and with regard for appropriate balance. This material is based on work supported by the National Science Foundation under Grant No. CTS- 0096624. Any opinions, findings, and conclusions or recommendations expressed in it are those of the authors and do not necessarily reflect the views of the National Science Foundation. International Standard Book Number 0-309-08454-7 Additional copies of this report are available from: National Research Council 2101 Constitution Avenue, N.W. Washington, DC 20418 Internet, <http://www.nap.edu> Copyright 2002 by the National Academy of Sciences. All rights reserved. Printed in the United States of America Front cover: Three-dimensional scanning tunneling microscope image of a man-made lattice of cobalt atoms on a copper (111) surface. Courtesy of Don Eigler, IBM Almaden Research Center. Back cover: A nanoscale motor created by attaching a synthetic rotor to an ATP synthase. Reprinted with permission of the American Association for the Advancement of Science from Soong et al., Science 290, 1555 (2000). © 2000 by AAAS. -
ABSTRACT MARTIN, KELLY NORRIS. Visual
ABSTRACT MARTIN, KELLY NORRIS. Visual Research: Introducing a Schema for Methodologies and Contexts. (Under the direction of Victoria J. Gallagher.) Studying the visual has become tremendously important to many disciplines because images express a range of human experience sometimes ambiguously articulated in verbal discourse, namely, “spatially oriented, nonlinear, multidimensional, and dynamic” human experiences (Foss, 2005, p. 143). In fact, the power of the image to plainly communicate occurs not “in spite of language’s absence but also frequently because of language’s absence” (Ott & Dickinson, 2009, p. 392). Presently, the challenge for visual research is that scholars investigate images from varied disciplines with separate and distinctive methods with little discussion or exchange across fields. Furthermore, more disciplines are requiring students to take courses in visual communication and more professors are being hired to teach those courses. However, these visual communication professors have nowhere to go (in the United States) in order to become prepared to teach and conduct research in visual communication. They enroll in programs in journalism and mass communication, linguistics, sociology, social psychology, anthropology, and so on. Then, they either adapt what they learn from these fields to the field of visual communication or they teach themselves about the methods, theories, and literature of visual communication. This project focused on comparing and contrasting the strengths and limitations of various visual research -
Dr Don Eigler Hon Dsc
Dr Don Eigler Hon DSc Oration by Professor Chris McConville Department of Physics Dr Don Eigler Hon DSc I am personally delighted that today’s honorary graduate is being given to study non-interacting inert gas atoms such as xenon on a metal surface. this award. Dr Don Eigler is quite literally a giant in the “Small World” of However, he observed that even at these low temperatures the Xe atoms Nanoscience and Nanotechnology, and he is universally acknowledged as would still change positions on the surface due to the forces exerted on the first person ever to move and control a single atom. them by the tip of the microscope. He concluded that if these forces could be controlled, he should be able to move the atoms deliberately. Nanotechnology impacts on all our lives, from the ever-present smartphone to medical and environmental applications, but its origins On 10th November 1989 he arranged 35 xenon atoms on a nickel surface can be said to have begun in December 1959 with a lecture by the to spell out ‘I.B.M.’ As he said in his log book this was “the first ever visionary – and often controversial – physicist, Richard Feynman, entitled construction of a patterned array of atoms”. That now famous image not There’s Plenty of Room at the Bottom. In it Feynman speculated that, “The only appeared on the front cover of Nature, but when the news broke in an principles of physics, as far as I can see, do not speak against the possibility IBM press release, on the front page of most broadsheet newspapers in of maneuvering things atom by atom, arranging them the way we want the western world. -
Electronic Transport in Single-Walled Carbon Nanotubes, and Their Application As Scanning Probe Microscopy Tips
Electronic Transport in Single-Walled Carbon Nanotubes, and their Application as Scanning Probe Microscopy tips by Neil Richard Wilson Thesis Submitted to the University of Warwick for the degree of Doctor of Philosophy Physics April 2004 ii Contents List of Tables vii List of Figures viii Acknowledgments xiv Declarations xvi Abstract xviii Abbreviations xix Chapter 1 Introduction 1 1.1 Introduction to Single-Walled Carbon Nanotubes ........... 3 History .............................. 4 Structure and mechanical properties .............. 6 Electronic properties of SWNT ................. 9 Why study SWNT devices: the Physics and the Funding . 17 1.2 Introduction to Atomic Force Microscopy . 20 Dynamic or 'Tapping' mode AFM . 24 Dynamic lateral force mode or 'Torsional Resonance' mode . 30 Electric Force Microscopy .................... 33 iii Tips and Cantilevers ....................... 36 Multimode and Dimension AFM's . 39 1.3 Outline of thesis ............................. 41 Chapter 2 SWNT growth and devices 42 2.1 SWNT growth .............................. 42 2.1.1 Experimental setup and results . 44 2.1.2 Characterisation ......................... 53 AFM and SCM .......................... 53 Electron Microscopy ....................... 55 micro-Raman spectroscopy ................... 59 2.2 SWNT devices .............................. 62 Lithography ............................ 63 Device Fabrication ........................ 66 2.2.1 Room Temperature Electronic Transport Characteristics . 68 2.3 Conclusions and future work ...................... 75 Chapter 3 EFM and SGM of carbon nanotube devices 77 3.1 Experimental setup for EFM and SGM . 79 3.2 Manipulation of SWNT devices, and characterisation by SGM . 82 3.3 SSPM of SWNT devices ......................... 89 3.3.1 Current saturation in mSWNT devices . 90 3.3.2 Hysteresis in the transconductance of SWNT devices . 97 3.4 Conclusions and future work . 101 Chapter 4 SWNT as AFM probes 103 4.1 Fabrication of SWNT-AFM tips . -
Sustainable Development for The
“When I look at astronauts … buzzing around SPECIAL “[Space debris] models don’t scaffolding … I want to know who provided 2018 GALA INSERT predict the future, they ... predict the the worker’s comp.” p7 Celebrate the Extraordinary! most likely future.” p14 THE NEW YORK ACADEMY OF CIENCES SMAGAZINE • FALL 2018 BEYOND 2030: SUSTAINABLE DEVELOPMENT FOR THE A look at the sustainability challenges of future human space exploration WWW.NYAS.ORG BOARD OF GOVERNORS CHAIR SECRETARY Beth Jacobs, Managing Lowell Robinson,a highly INTERNATIONAL Paul Stoffels, Vice Chair of Paul Horn, Former Senior Larry Smith, The New York Partner of Excellentia regarded executive with BOARD OF GOVERNORS the Executive Committee Vice Provost for Research, Academy of Sciences Global Partners thirty years of senior global Seth F. Berkley, Chief and Chief Scientific Officer, New York University strategic, financial, M&A, Executive Officer, The Johnson & Johnson GOVERNORS John E. Kelly III, SVP, Senior Vice Dean for operational, turnaround and Global Alliance for Vaccines Ellen de Brabander, Senior Solutions Portfolio and CHAIRS EMERITI Strategic Initiatives and governance experience at and Immunization Vice President Research Research, IBM John E. Sexton, Former Entrepreneurship, NYU both Fortune 100 consumer and Development Global Seema Kumar, Vice Stefan Catsicas, Chief Tech- President, New York Polytechnic School of products retail and diversi- Functions, Governance & President of Innovation, nology Officer Nestlé S.A. University Engineering fied financial services Compliance, PepsiCo Global Health and Science Gerald Chan, Co-Founder, Torsten N. Wiesel, Kathe Sackler, Founder VICE-CHAIR Jacqueline Corbelli, Policy Communication for Morningside Group Nobel Laureate & former and President, The Acorn Thomas Pompidou, Chairman, CEO and Johnson & Johnson Alice P. -
An Overview of the State of Chinese Nanoscience and Technology
SMALL SCIENCE IN BIG CHINA An overview of the state of Chinese nanoscience and technology. Conducted in collaboration between Springer Nature, the National Center for Nanoscience and Technology, China, and the National Science Library of the Chinese Academy of Sciences. Ed Gerstner The National Center for Nanoscience and Springer Nature, China Minghua Liu Technology, China National Center for The National Center for Nanoscience and Technology, China (NCNST) was established in December 2003 by the Nanoscience and Chinese Academy of Science (CAS) and the Ministry of Education as an institution dedicated to fundamental and Technology, China applied research in the field of nanoscience and technology, especially those with important potential applications. Xiangyang Huang NCNST is operated under the supervision of the Governing Board and aims to become a world-class research National Science Library, centre, as well as public technological platform and young talents training centre in the field, and to act as an Chinese Academy of important bridge for international academic exchange and collaboration. Sciences The NCNST currently has three CAS Key Laboratories: the CAS Key Laboratory for Biological Effects of Yingying Zhou Nanomaterials & Nanosafety, the CAS Key Laboratory for Standardization & Measurement for Nanotechnology, Nature Research, Springer and the CAS Key Laboratory for Nanosystem and Hierarchical Fabrication. Besides, there is a division of Nature, China nanotechnology development, which is responsible for managing the opening and sharing of up-to-date instruments and equipment on the platform. The NCNST has also co-founded 19 collaborative laboratories with Zhiyong Tang Tsinghua University, Peking University, and CAS. National Center for The NCNST has doctoral and postdoctoral education programs in condensed matter physics, physical Nanoscience and chemistry, materials science, and nanoscience and technology. -
Photonic Crystals
Velkommen I Nanoskolen blir du kjent med nanomaterialer i form av partikler, tråder, filmer og faste materialer. Du lærer også om biologiske nanomaterialer og bruk i medisin, samt hvordan du kan få energi fra nanostrukturer. Timeplan MANDAG TIRSDAG ONSDAG TORSDAG FREDAG Start 8:30: Mottak, Gruppe 1: Gruppe 2: Gruppe 1: Gruppe 2: Gruppe 1: Gruppe 2: ALLE: registrering, beskjeder (Berzelius) Lab 1: Forelesning: Lab 2: Forelesning: Lab 3: Forelesning: Programmerings 9.00 – 9.30 Velkommen, info Nanopartikler Nano med Overflater Solceller med Spesielle Bionano med -teori med 09:00- 9.30 – 10:30 Bli-kjent leker Ola Torunn & egenskaper Elina (Curie) Haakon 11:30 10:30 – 10:45 Pause + Solcelle (Berzelius) Lasse (Berzelius) 10:45 – 11:30 Labboka og + Solcelle + Solcelle Forelesning: intro til Nano Forelesning: Nano med Programmering Nano med Ola med Arduino Ola 11:30- Lunsj / Utelek Lunsj / Utelek Lunsj / Utelek Lunsj / Utelek Lunsj / Utelek 12:30 12:30-12:45 Felles gange til Gruppe 1: Gruppe 2: Gruppe 1: Gruppe 2: Gruppe 1: Gruppe 2: ALLE: Forskningsparken/MiNa 12:45-13:45 MiNa/FP (De Forelesning: Lab 1: Forelesning: Lab 2: Forelesning: Lab 3: Programmering deles inn i grupper på hvert Nano med Nanopartikler Solceller med Overflater Bionano med Spesielle med Arduino sted som får hver sin Ola Torunn & Elina (Curie) egenskaper 12:30- omvisning) (Berzelius) + Solcelle Lasse + Solcelle 15:00 13:45-14:00 Bytte sted: + Solcelle Avslutning og MiNa/FP Forelesning: evaluering. 14:00-15:00 FP/MiNa (De Nano med deles inn i grupper på hvert Ola sted som -
Representation in Scientific Practice Revisited Inside Technology Edited by Wiebe E
Representation in Scientific Practice Revisited Inside Technology edited by Wiebe E. Bijker, W. Bernard Carlson, and Trevor Pinch A list of books in the series appears at the back of the book. Representation in Scientific Practice Revisited edited by Catelijne Coopmans, Janet Vertesi, Michael Lynch, and Steve Woolgar The MIT Press Cambridge, Massachusetts London, England © 2014 Massachusetts Institute of Technology All rights reserved. No part of this book may be reproduced in any form by any electronic or me- chanical means (including photocopying, recording, or information storage and retrieval) with- out permission in writing from the publisher. MIT Press books may be purchased at special quantity discounts for business or sales promotional use. For information, please email [email protected]. This book was set in Stone Sans and Stone Serif by Toppan Best-set Premedia Limited, Hong Kong. Printed and bound in the United States of America. Library of Congress Cataloging-in-Publication Data Representation in scientifi c practice revisited / edited by Catelijne Coopmans, Janet Vertesi, Michael Lynch, and Steve Woolgar. pages cm. — (Inside technology) Includes bibliographical references and index. ISBN 978-0-262-52538-1 (pbk. : alk. paper) 1. Research — Methodology. 2. Science — Methodology. 3. Technology — Methodology. I. Coopmans, Catelijne, 1976 – editor of compilation. Q180.55.M4R455 2014 502.2 ′ 2 — dc23 2013014968 10 9 8 7 6 5 4 3 2 1 Contents Preface vii Michael Lynch and Steve Woolgar 1 Introduction: Representation in Scientific Practice Revisited 1 Catelijne Coopmans, Janet Vertesi, Michael Lynch, and Steve Woolgar Chapters 2 Drawing as : Distinctions and Disambiguation in Digital Images of Mars 15 Janet Vertesi 3 Visual Analytics as Artful Revelation 37 Catelijne Coopmans 4 Digital Scientific Visuals as Fields for Interaction 61 Morana Alač 5 Swimming in the Joint 89 Rachel Prentice 6 Chalk: Materials and Concepts in Mathematics Research 107 Michael J. -
Richard Feynman's Famous Talk on Atom-By-Atom Assembly Is Often
Nanotechnology Feynman’s fancy Richard Feynman’s famous talk on atom-by-atom assembly is often credited with kick-starting nanotechnology. Fifty years on, Philip Ball investigates how influential it really was KEVIN FLEMING / CORBIS / FLEMING KEVIN 58 | Chemistry World | January 2009 www.chemistryworld.org Fifty years ago, the near-legendary might be wise to stop pretending microscope (STM) to manipulate In short physicist Richard Feynman of the that his address to the APS was individual xenon atoms. These were California Institute of Technology the ‘birth of nanotechnology,’ that Nobel prize winner adsorbed on the surface of nickel, (Caltech) gave a talk called There’s needn’t prevent us from relishing the Richard Feynman is often creating letters five atoms high and plenty of room at the bottom to spectacle of a genius giving free rein linked to the ‘birth of achieving a data storage density over the American Physical Society’s to his imagination. It was, according nanotechnology’ 100 times greater than Feynman’s West Coast section. He outlined a to George Whitesides of Harvard Fifty years ago, conservative estimate for what might vision of what would later be called University, US, ‘yet more validation, Feynman gave an be needed to write with atoms. nanotechnology, imagining ‘that we if any were needed, of Feynman’s imaginative talk outlining Indeed, one could say that could arrange atoms one by one, just perceptiveness and openness to new a nano vision, where Feynman didn’t think small enough. as we want them’. The rest is history. -
Part 3 Proceedings of the Roadmap Working Group
Part 3 Proceedings of the Roadmap Working Group The Roadmap development process included four intensive workshops held from October 2005 through December 2006. Following an inaugural workshop San Francisco, the meetings were sponsored by the Battelle Memorial Foundation and hosted by the Oak Ridge, Brookhaven, and Pacific Northwest National Laboratories. The Working Group Proceedings presents a collection of papers, extended abstracts, and personal perspectives contributed by participants in the Roadmap workshops and subsequent online exchanges. These contributions are included with the Roadmap document to make available, to the extent possible, the full range of ideas and information brought to the Roadmap process by its participants. The papers are arranged in the order given below. Atomically Precise Fabrication 01 Atomically Precise Manufacturing Processes 02 Mechanosynthesis 03 Patterned ALE Path Phases 04 Numerically Controlled Molecular Epitaxy 05 Scanning Probe Diamondoid Mechanosynthesis 06 Limitations of Bottom-Up Assembly 07 Nucleic Acid Engineering 08 DNA as an Aid to Self-Assembly 09 Self-Assembly 10 Protein Bioengineering Overview 11 Synthetic Chemistry 12 A Path to a Second Generation Nanotechnology 13 Atomically Precise Ceramic Structures 14 Enabling Nanoscience for Atomically-Precise Manufacturing of Functional Nanomaterials Nanoscale Structures and Fabrication 15 Lithography and Applications of New Nanotechnology 16 Scaling Up to Large Production of Nanostructured Materials Nanotechnology Roadmap Working Group Proceedings -
Nanotechnology and Innovation Policy
Harvard Journal of Law & Technology Volume 29, Number 1 Fall 2015 NANOTECHNOLOGY AND INNOVATION POLICY Lisa Larrimore Ouellette* TABLE OF CONTENTS I. INTRODUCTION ................................................................................ 34 II. NANOTECHNOLOGY’S DEVELOPMENT AND ECONOMIC CONTRIBUTION ............................................................................... 37 A. Selected Developments in Nanotechnology ................................ 38 1. Research Tools: Seeing at the Nanoscale ................................ 39 2. Promising Nanomaterials: Fullerenes, Nanotubes, and Graphene ........................................................................... 42 3. Commercial Nanoelectronics .................................................. 46 B. Nanotechnology’s Economic Contribution ................................ 47 1. Qualitative Analysis of Nanotechnology’s Transformative Potential ................................................... 47 2. Quantitative Estimates of the Nanotechnology Market ........... 48 III. IP AND NANOTECHNOLOGY .......................................................... 51 A. Patents ........................................................................................ 52 1. Potential Limitations on the Patentability of Nanotechnology ................................................................ 52 2. Knowledge Diffusion Through Patent Disclosure................... 54 3. Patent Thickets and Patent Litigation ...................................... 56 B. Trade Secrets .............................................................................