Towards an Understanding of Symbiont Natural History Through Studies Of
Total Page:16
File Type:pdf, Size:1020Kb
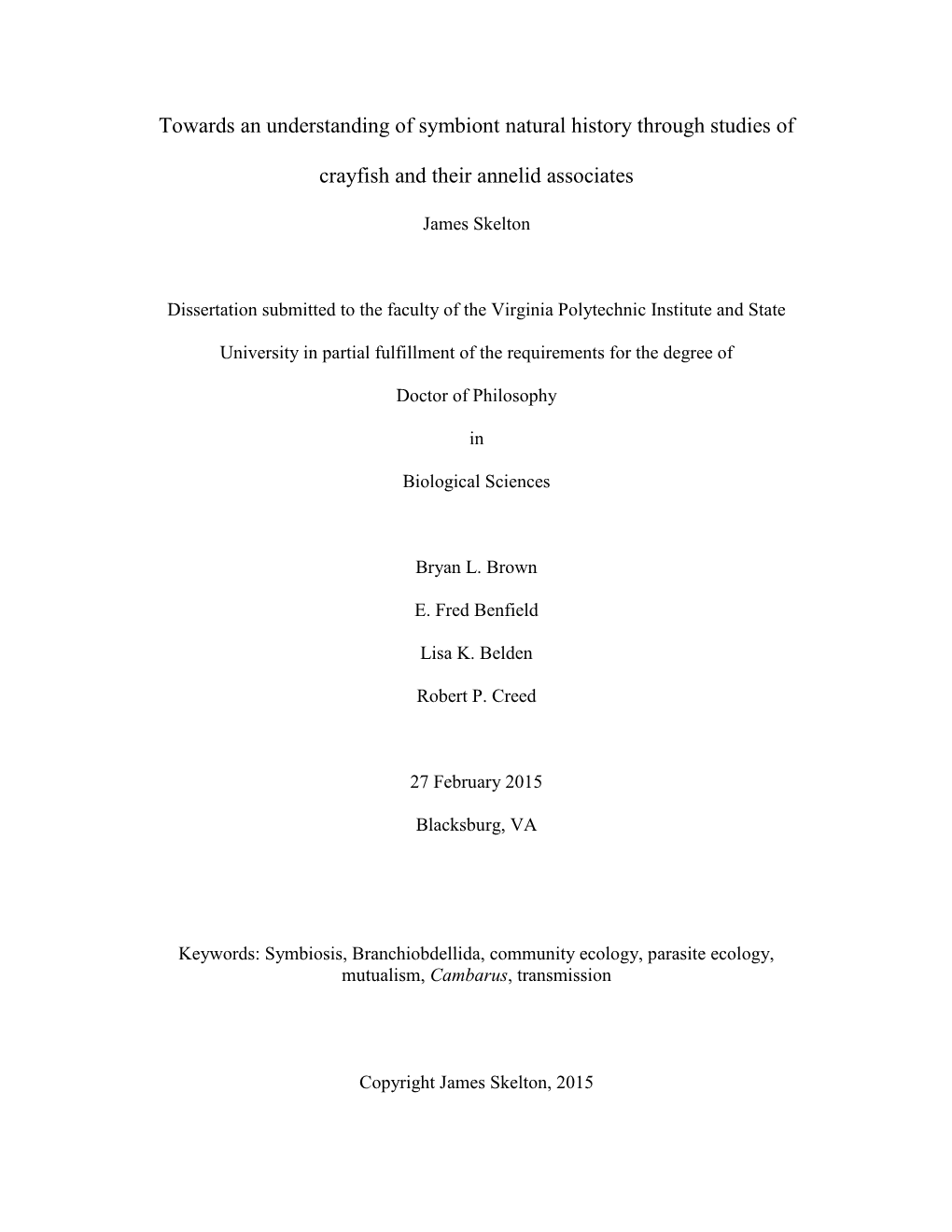
Load more
Recommended publications
-
New Alien Crayfish Species in Central Europe
NEW ALIEN CRAYFISH SPECIES IN CENTRAL EUROPE Introduction pathways, life histories, and ecological impacts DISSERTATION zur Erlangung des Doktorgrades Dr. rer. nat. der Fakultät für Naturwissenschaften der Universität Ulm vorgelegt von Christoph Chucholl aus Rosenheim Ulm 2012 NEW ALIEN CRAYFISH SPECIES IN CENTRAL EUROPE Introduction pathways, life histories, and ecological impacts DISSERTATION zur Erlangung des Doktorgrades Dr. rer. nat. der Fakultät für Naturwissenschaften der Universität Ulm vorgelegt von Christoph Chucholl aus Rosenheim Ulm 2012 Amtierender Dekan: Prof. Dr. Axel Groß Erstgutachter: Prof. Dr. Manfred Ayasse Zweitgutachter: Prof. apl. Dr. Gerhard Maier Tag der Prüfung: 16.7.2012 Cover picture: Orconectes immunis male (blue color morph) (photo courtesy of Dr. H. Bellmann) Table of contents Part 1 – Summary Introduction ............................................................................................................................ 1 Invasive alien species – a global menace ....................................................................... 1 “Invasive” matters .......................................................................................................... 2 Crustaceans – successful invaders .................................................................................. 4 The case of alien crayfish in Europe .............................................................................. 5 New versus Old alien crayfish ....................................................................................... -
Evolutionary History of Inversions in the Direction of Architecture-Driven
bioRxiv preprint doi: https://doi.org/10.1101/2020.05.09.085712; this version posted May 10, 2020. The copyright holder for this preprint (which was not certified by peer review) is the author/funder, who has granted bioRxiv a license to display the preprint in perpetuity. It is made available under aCC-BY-NC 4.0 International license. Evolutionary history of inversions in the direction of architecture- driven mutational pressures in crustacean mitochondrial genomes Dong Zhang1,2, Hong Zou1, Jin Zhang3, Gui-Tang Wang1,2*, Ivan Jakovlić3* 1 Key Laboratory of Aquaculture Disease Control, Ministry of Agriculture, and State Key Laboratory of Freshwater Ecology and Biotechnology, Institute of Hydrobiology, Chinese Academy of Sciences, Wuhan 430072, China. 2 University of Chinese Academy of Sciences, Beijing 100049, China 3 Bio-Transduction Lab, Wuhan 430075, China * Corresponding authors Short title: Evolutionary history of ORI events in crustaceans Abbreviations: CR: control region, RO: replication of origin, ROI: inversion of the replication of origin, D-I skew: double-inverted skew, LBA: long-branch attraction bioRxiv preprint doi: https://doi.org/10.1101/2020.05.09.085712; this version posted May 10, 2020. The copyright holder for this preprint (which was not certified by peer review) is the author/funder, who has granted bioRxiv a license to display the preprint in perpetuity. It is made available under aCC-BY-NC 4.0 International license. Abstract Inversions of the origin of replication (ORI) of mitochondrial genomes produce asymmetrical mutational pressures that can cause artefactual clustering in phylogenetic analyses. It is therefore an absolute prerequisite for all molecular evolution studies that use mitochondrial data to account for ORI events in the evolutionary history of their dataset. -
Tc & Forward & Owls-I-IX
USDA Forest Service 1997 General Technical Report NC-190 Biology and Conservation of Owls of the Northern Hemisphere Second International Symposium February 5-9, 1997 Winnipeg, Manitoba, Canada Editors: James R. Duncan, Zoologist, Manitoba Conservation Data Centre Wildlife Branch, Manitoba Department of Natural Resources Box 24, 200 Saulteaux Crescent Winnipeg, MB CANADA R3J 3W3 <[email protected]> David H. Johnson, Wildlife Ecologist Washington Department of Fish and Wildlife 600 Capitol Way North Olympia, WA, USA 98501-1091 <[email protected]> Thomas H. Nicholls, retired formerly Project Leader and Research Plant Pathologist and Wildlife Biologist USDA Forest Service, North Central Forest Experiment Station 1992 Folwell Avenue St. Paul, MN, USA 55108-6148 <[email protected]> I 2nd Owl Symposium SPONSORS: (Listing of all symposium and publication sponsors, e.g., those donating $$) 1987 International Owl Symposium Fund; Jack Israel Schrieber Memorial Trust c/o Zoological Society of Manitoba; Lady Grayl Fund; Manitoba Hydro; Manitoba Natural Resources; Manitoba Naturalists Society; Manitoba Critical Wildlife Habitat Program; Metro Propane Ltd.; Pine Falls Paper Company; Raptor Research Foundation; Raptor Education Group, Inc.; Raptor Research Center of Boise State University, Boise, Idaho; Repap Manitoba; Canadian Wildlife Service, Environment Canada; USDI Bureau of Land Management; USDI Fish and Wildlife Service; USDA Forest Service, including the North Central Forest Experiment Station; Washington Department of Fish and Wildlife; The Wildlife Society - Washington Chapter; Wildlife Habitat Canada; Robert Bateman; Lawrence Blus; Nancy Claflin; Richard Clark; James Duncan; Bob Gehlert; Marge Gibson; Mary Houston; Stuart Houston; Edgar Jones; Katherine McKeever; Robert Nero; Glenn Proudfoot; Catherine Rich; Spencer Sealy; Mark Sobchuk; Tom Sproat; Peter Stacey; and Catherine Thexton. -
New River Crayfish Range Wide Status Assessment
New River Crayfish Range Wide Status Assessment William T. Russ, North Carolina Wildlife Resources Commission, Division of Inland Fisheries, 645 Fish Hatchery Road, Marion, NC 28752 Zach J. Loughman, West Liberty University, Department of Natural Sciences and Mathematics, Campus Service Center Box 139, West Liberty University, West Liberty, WV 26074 Roger F. Thoma, Midwest Biodiversity Institute, Inc., 4673 Northwest Parkway, Hilliard, OH 43026 Brian T. Watson, Virginia Department of Game and Inland Fisheries, 1132 Thomas Jefferson Road, Forest, VA 24551 Todd D. Ewing, North Carolina Wildlife Resources Commission, Division of Inland Fisheries, 1721 Mail Service Center, Raleigh, NC 27699 Abstract: The New River crayfish, (Cambarus chasmodactylus), was described in 1966 from the East Fork of the Greenbrier River, West Virginia, and historically occurred throughout the New River Basin from the Greenbrier River sub-basin in West Virginia, upstream through Virginia, and into the headwaters of the South Fork New River in North Carolina. The New River crayfish was part of a federal listing species petition in 2010 and it is cur- rently being evaluated for listing as either threatened or endangered by the U.S. Fish and Wildlife Service under the Endangered Species Act. In order to understand the current distribution and status of this species, a range-wide assessment was undertaken by various organizations and agencies in West Virginia, Virginia, and North Carolina. Biological information was summarized, including species description, habitat use, life history, and current distribution. All historical and recent collections were compared and spatially displayed using GIS software. The New River crayfish was collected in three 8-digit hydrologic unit codes (HUCs) and 14 counties in three states, with the majority of occurrences in the Upper New and Greenbrier River sub-basins. -
South Carolina Department of Natural Resources
FOREWORD Abundant fish and wildlife, unbroken coastal vistas, miles of scenic rivers, swamps and mountains open to exploration, and well-tended forests and fields…these resources enhance the quality of life that makes South Carolina a place people want to call home. We know our state’s natural resources are a primary reason that individuals and businesses choose to locate here. They are drawn to the high quality natural resources that South Carolinians love and appreciate. The quality of our state’s natural resources is no accident. It is the result of hard work and sound stewardship on the part of many citizens and agencies. The 20th century brought many changes to South Carolina; some of these changes had devastating results to the land. However, people rose to the challenge of restoring our resources. Over the past several decades, deer, wood duck and wild turkey populations have been restored, striped bass populations have recovered, the bald eagle has returned and more than half a million acres of wildlife habitat has been conserved. We in South Carolina are particularly proud of our accomplishments as we prepare to celebrate, in 2006, the 100th anniversary of game and fish law enforcement and management by the state of South Carolina. Since its inception, the South Carolina Department of Natural Resources (SCDNR) has undergone several reorganizations and name changes; however, more has changed in this state than the department’s name. According to the US Census Bureau, the South Carolina’s population has almost doubled since 1950 and the majority of our citizens now live in urban areas. -
Natural Heritage Program List of Rare Animal Species of North Carolina 2018
Natural Heritage Program List of Rare Animal Species of North Carolina 2018 Carolina Northern Flying Squirrel (Glaucomys sabrinus coloratus) photo by Clifton Avery Compiled by Judith Ratcliffe, Zoologist North Carolina Natural Heritage Program N.C. Department of Natural and Cultural Resources www.ncnhp.org C ur Alleghany rit Ashe Northampton Gates C uc Surry am k Stokes P d Rockingham Caswell Person Vance Warren a e P s n Hertford e qu Chowan r Granville q ot ui a Mountains Watauga Halifax m nk an Wilkes Yadkin s Mitchell Avery Forsyth Orange Guilford Franklin Bertie Alamance Durham Nash Yancey Alexander Madison Caldwell Davie Edgecombe Washington Tyrrell Iredell Martin Dare Burke Davidson Wake McDowell Randolph Chatham Wilson Buncombe Catawba Rowan Beaufort Haywood Pitt Swain Hyde Lee Lincoln Greene Rutherford Johnston Graham Henderson Jackson Cabarrus Montgomery Harnett Cleveland Wayne Polk Gaston Stanly Cherokee Macon Transylvania Lenoir Mecklenburg Moore Clay Pamlico Hoke Union d Cumberland Jones Anson on Sampson hm Duplin ic Craven Piedmont R nd tla Onslow Carteret co S Robeson Bladen Pender Sandhills Columbus New Hanover Tidewater Coastal Plain Brunswick THE COUNTIES AND PHYSIOGRAPHIC PROVINCES OF NORTH CAROLINA Natural Heritage Program List of Rare Animal Species of North Carolina 2018 Compiled by Judith Ratcliffe, Zoologist North Carolina Natural Heritage Program N.C. Department of Natural and Cultural Resources Raleigh, NC 27699-1651 www.ncnhp.org This list is dynamic and is revised frequently as new data become available. New species are added to the list, and others are dropped from the list as appropriate. The list is published periodically, generally every two years. -
The Crayfishes of West Virginia's Southwestern Coalfields Region
Marshall University Marshall Digital Scholar Theses, Dissertations and Capstones 1-1-2013 The rC ayfishes of West Virginia’s Southwestern Coalfields Region with an Emphasis on the Life History of Cambarus theepiensis David Allen Foltz II Follow this and additional works at: http://mds.marshall.edu/etd Part of the Aquaculture and Fisheries Commons, and the Ecology and Evolutionary Biology Commons Recommended Citation Foltz, David Allen II, "The rC ayfishes of West Virginia’s Southwestern Coalfields Region with an Emphasis on the Life History of Cambarus theepiensis" (2013). Theses, Dissertations and Capstones. Paper 731. This Thesis is brought to you for free and open access by Marshall Digital Scholar. It has been accepted for inclusion in Theses, Dissertations and Capstones by an authorized administrator of Marshall Digital Scholar. For more information, please contact [email protected]. The Crayfishes of West Virginia’s Southwestern Coalfields Region with an Emphasis on the Life History of Cambarus theepiensis A Thesis submitted to the Graduate College of Marshall University Huntington, WV In partial fulfillment of the requirements for the degree of Master of Science Biological Sciences: Watershed Resource Science Prepared by David Allen Foltz II Approved by Committee Members: Zachary Loughman, Ph.D., Major Advisor David Mallory, Ph.D., Committee Member Mindy Armstead, Ph.D., Committee Member Thomas Jones, Ph.D., Committee Member Thomas Pauley, Ph.D., Committee Member Marshall University Defended 11/13/2013 Final Submission to the Graduate College December 2013 ©2013 David Allen Foltz II ALL RIGHTS RESERVED ii AKNOWLEDGMENTS I would like to extend my gratitude to my committee members. -
At-Risk Species Assessment on Southern National Forests, Refuges, and Other Protected Areas
David Moynahan | St. Marks NWR At-Risk Species Assessment on Southern National Forests, Refuges, and Other Protected Areas National Wildlife Refuge Association Mark Sowers, Editor October 2017 1001 Connecticut Avenue NW, Suite 905, Washington, DC 20036 • 202-417-3803 • www.refugeassociation.org At-Risk Species Assessment on Southern National Forests, Refuges, and Other Protected Areas Table of Contents Introduction and Methods ................................................................................................3 Results and Discussion ......................................................................................................9 Suites of Species: Occurrences and Habitat Management ...........................................12 Progress and Next Steps .................................................................................................13 Appendix I: Suites of Species ..........................................................................................17 Florida Panhandle ............................................................................................................................18 Peninsular Florida .............................................................................................................................28 Southern Blue Ridge and Southern Ridge and Valley ...............................................................................................................................39 Interior Low Plateau and Cumberland Plateau, Central Ridge and Valley ...............................................................................................46 -
Synopsis of Freshwater Crayfish Diseases and Commensal Organisms Brett .F Edgerton James Cook University, [email protected]
University of Nebraska - Lincoln DigitalCommons@University of Nebraska - Lincoln Faculty Publications from the Harold W. Manter Parasitology, Harold W. Manter Laboratory of Laboratory of Parasitology 3-2002 Synopsis of Freshwater Crayfish Diseases and Commensal Organisms Brett .F Edgerton James Cook University, [email protected] Louis H. Evans Curtin University of Technology Frances J. Stephens Curtin University of Technology Robin M. Overstreet Gulf Coast Research Laboratory, [email protected] Follow this and additional works at: https://digitalcommons.unl.edu/parasitologyfacpubs Part of the Aquaculture and Fisheries Commons, and the Parasitology Commons Edgerton, Brett .;F Evans, Louis H.; Stephens, Frances J.; and Overstreet, Robin M., "Synopsis of Freshwater Crayfish Diseases and Commensal Organisms" (2002). Faculty Publications from the Harold W. Manter Laboratory of Parasitology. 884. https://digitalcommons.unl.edu/parasitologyfacpubs/884 This Article is brought to you for free and open access by the Parasitology, Harold W. Manter Laboratory of at DigitalCommons@University of Nebraska - Lincoln. It has been accepted for inclusion in Faculty Publications from the Harold W. Manter Laboratory of Parasitology by an authorized administrator of DigitalCommons@University of Nebraska - Lincoln. Published in Aquaculture 206:1–2 (March 2002), pp. 57–135; doi: 10.1016/S0044-8486(01)00865-1 Copyright © 2002 Elsevier Science. Creative Commons Attribution Non-Commercial No Deriva- tives License. Accepted October 18, 2001; published online November 30, 2001. Synopsis of Freshwater Crayfish Diseases and Commensal Organisms Brett F. Edgerton,1 Louis H. Evans,2 Frances J. Stephens,2 and Robin M. Overstreet3 1. Department of Microbiology and Immunology, James Cook University, Townsville, QLD 4810, Australia 2. -
NEPA--Environmental Impact Statement
United States Final Environmental Impact Department of Agriculture Statement Forest Service May 20, 2013 AP Loblolly Pine Removal and Restoration Project Andrew Pickens Ranger District, Sumter National Forest Oconee County, South Carolina Final Environmental Impact Statement AP Loblolly Pine Removal and Restoration Project AP Loblolly Pine Removal and Restoration Project Final Environmental Impact Statement Oconee County, South Carolina Lead Agency: USDA Forest Service Responsible Official: MICHAEL B. CRANE, DISTRICT RANGER 112 Andrew Pickens Circle Mountain Rest, SC 29664 For Information Contact: VICTOR WYANT, SILVICULTURIST 112 Andrew Pickens Circle Mountain Rest, SC 29664 864-638-9568 Abstract: The objective of this project is to restore native vegetation typical of the Southern Appalachian Mountains by removing non-native loblolly pine plantations. This would improve ecosystem health and increase habitat diversity. The majority of the project area (77%) falls within the Shortleaf Pine-Oak Ecological Zone, which in the absence of loblolly pine would consist of shortleaf pine and oak dominated forests in the lower elevation Southern Appalachians. Examples of this zone can occur on a variety of topographic and landscape positions, including ridge tops, upper and mid-slopes, as well as low elevation mountain valleys. Pitch pine (and Table Mountain pine) may sometimes be present; Virginia pine and hardwoods are sometimes abundant, especially dry- site oaks such as southern red oak, post oak, blackjack oak, chestnut oak, scarlet oak, but also pignut hickory, red maple, and other associated species. Frequent, low-intensity fires, coupled with severe fires, can influence the occurrence of pines in this zone, particularly shortleaf pine, pitch pine, or Table Mountain pine, rather than hardwood forests or Virginia pine (Natureserve, 2011). -
Sensitive Species That Are Not Listed Or Proposed Under the ESA Sorted By: Major Group, Subgroup, NS Sci
Forest Service Sensitive Species that are not listed or proposed under the ESA Sorted by: Major Group, Subgroup, NS Sci. Name; Legend: Page 94 REGION 10 REGION 1 REGION 2 REGION 3 REGION 4 REGION 5 REGION 6 REGION 8 REGION 9 ALTERNATE NATURESERVE PRIMARY MAJOR SUB- U.S. N U.S. 2005 NATURESERVE SCIENTIFIC NAME SCIENTIFIC NAME(S) COMMON NAME GROUP GROUP G RANK RANK ESA C 9 Anahita punctulata Southeastern Wandering Spider Invertebrate Arachnid G4 NNR 9 Apochthonius indianensis A Pseudoscorpion Invertebrate Arachnid G1G2 N1N2 9 Apochthonius paucispinosus Dry Fork Valley Cave Invertebrate Arachnid G1 N1 Pseudoscorpion 9 Erebomaster flavescens A Cave Obligate Harvestman Invertebrate Arachnid G3G4 N3N4 9 Hesperochernes mirabilis Cave Psuedoscorpion Invertebrate Arachnid G5 N5 8 Hypochilus coylei A Cave Spider Invertebrate Arachnid G3? NNR 8 Hypochilus sheari A Lampshade Spider Invertebrate Arachnid G2G3 NNR 9 Kleptochthonius griseomanus An Indiana Cave Pseudoscorpion Invertebrate Arachnid G1 N1 8 Kleptochthonius orpheus Orpheus Cave Pseudoscorpion Invertebrate Arachnid G1 N1 9 Kleptochthonius packardi A Cave Obligate Pseudoscorpion Invertebrate Arachnid G2G3 N2N3 9 Nesticus carteri A Cave Spider Invertebrate Arachnid GNR NNR 8 Nesticus cooperi Lost Nantahala Cave Spider Invertebrate Arachnid G1 N1 8 Nesticus crosbyi A Cave Spider Invertebrate Arachnid G1? NNR 8 Nesticus mimus A Cave Spider Invertebrate Arachnid G2 NNR 8 Nesticus sheari A Cave Spider Invertebrate Arachnid G2? NNR 8 Nesticus silvanus A Cave Spider Invertebrate Arachnid G2? NNR -
Board of Game and Inland Fisheries Meeting Agenda
Revised Board of Game and Inland Fisheries 4000 West Broad Street, Board Room Richmond, Virginia 23230 August 14, 2012 9:00am Call to order and welcome, reading of the Mission Statement and Pledge of Allegiance to the Flag. 1. Recognition of Employees and Others 2. Public Comments – Department plan to build a new headquarters under PPEA 3. Public Comments – Non-Agenda Items 4. Approval of July 10, 2012 Board Meeting Minutes 5. Committee Meeting Reports: Wildlife, Boat and Law Enforcement Committee: Mr. Turner, Chairman of the Wildlife, Boat and Law Enforcement Committee, will report on the activities of the August 7, 2012 Committee Meeting. The Committee will recommend the following items to the full Board for final action: Staff Recommendations – Fisheries Regulation Amendments Staff Recommendations – Diversity Regulation Amendments Staff Recommendations – Boating Regulation Amendments Staff Recommendations – 2012-2013 Migratory Waterfowl Seasons and Bag Limits Staff Recommendations – ADA Regulation Agency Land Use Plan Proposed CY2013 Board Meeting Schedule Finance, Audit and Compliance Committee: Mr. Colgate, Chairman of the Finance, Audit and Compliance Committee, will report on the activities of the July 25, 2012 Committee Meeting. The Committee will present the following reports: FY2012 Year-end Financial Summary Internal Audit FY2013 Work Plan - Final Action Education, Planning and Outreach Committee: Ms. Caruso, Chairwoman of the Education, Planning, and Outreach Committee Meeting. Ms. Caruso will announce the next Committee Meeting will be held on October 17, 2012 beginning at 10:00am. 6. Closed Session 7. Director's Report: 8. Chairman's Remarks 9. Additional Business/Comments 10. Next Meeting Date: October 18, 2012 beginning at 9:00am 11.