Technological Assessment of Different Solar-Biomass Systems for Hybrid Power Generation in Europe
Total Page:16
File Type:pdf, Size:1020Kb
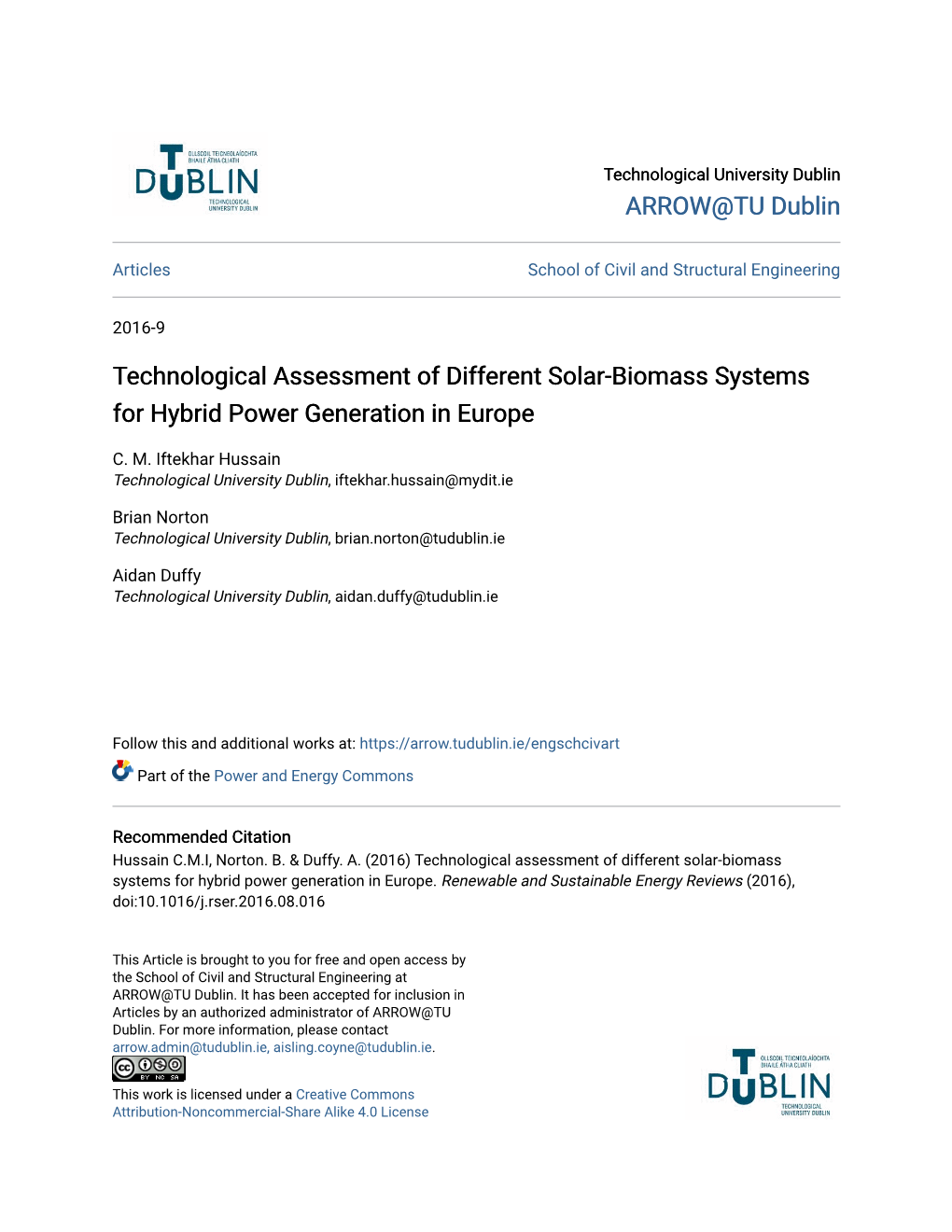
Load more
Recommended publications
-
PV Markets Spain & Portugal
PHOTOVOLTAICS IBERIA PV markets Spain & Portugal: Better than feared The PV farm Lo Illan, connected to the grid in the second Spain’s PV sector faces enormous challenges. The modification of the feed- quarter of 2011, is a project in tariff scheme and the economic crisis are seen as the main reasons. In of Gehrlicher Solar. Located in the Murcia province, the Portugal, the government has newly discovered PV and is now eager to get ground-mounted system has a total rated power of 2,065 kW. its piece of the cake. PV modules are made by First Solar, inverters by SMA. he conference “Conferencia de la Industria Spanish solar industry is able to compete on the glo- Photos (2): Gehrlicher Solar Solar-España 2011”, which took place in mid- bal stage, said Antonio Navarro of the European TOctober, reflected the situation of the Spanish Photovoltaic Industry Association (EPIA). solar sector – and it was said that it seems to be sur- According to the conference participants, the new prisingly good. In the opinion of Javier Anta of the target markets for the Spanish manufacturers will be Spanish Photovoltaic Industry Association ( Asociación mainly the United States, India, Australia, Latin de la Industria Fotovoltaica, ASIF), “the Spanish PV America and Morocco. They all agreed that the PV sector has shown that it is strong and steadfast even sector is in need of stability and a regulatory frame- under difficult external conditions including the un- work that will provide industry participants and in- stable regulatory framework and the economic cri- vestors with the necessary planning security. -
International Renewable Energy Entrepreneurship; a Mixed-Method
ADVERTIMENT. Lʼaccés als continguts dʼaquesta tesi queda condicionat a lʼacceptació de les condicions dʼús establertes per la següent llicència Creative Commons: http://cat.creativecommons.org/?page_id=184 ADVERTENCIA. El acceso a los contenidos de esta tesis queda condicionado a la aceptación de las condiciones de uso establecidas por la siguiente licencia Creative Commons: http://es.creativecommons.org/blog/licencias/ WARNING. The access to the contents of this doctoral thesis it is limited to the acceptance of the use conditions set by the following Creative Commons license: https://creativecommons.org/licenses/?lang=en International Renewable Energy Entrepreneurship; A Mixed- Method Approach By Seyed Meysam Zolfaghari Ejlal Manesh SUBMITTED TO THE DEPARTMENT OF BUSINESS IN PARTIAL FULLFILMENT OF THE REQUIRMENTS FOR THE DEGREE OF DOCTOR OF PHILOSOPHY IN ENTREPRENEURSHIP AND MANAGEMENT AT THE AUTONOMOUS UNIVERSITY OF BARCELONA (UAB) © MEYSAM ZOLFAGHARI .All rights reserved. Advisors: Dr. Alex Rialp Prof. Joaquim Vergés Barcelona November 2016 1 Abstract The demand for energy is increasing over time because of the rapid expansion of the global economy and population growth. However, conventional energy systems based on fossil fuels are not only an unreliable source of energy for the future but also cause a range of environmental consequences, including acidification, air pollution, global climate change, etc. Energy-based economic development (EBED) (Carley, Lawrence, Brown, Nourafshan, & Benami, 2011) and sustainable development (SD) (Hopwood, Mellor, & O’Brien, 2005) will consequently require a new source of energy based on renewable energies, which are more accessible, environmentally friendly, secure, and efficient. To address challenges associated with fossil fuels and fostering sustainable development, recent progress in the field of entrepreneurship has shown increased interest in sustainability issues and environmentally friendly technological development. -
CSP Technologies
CSP Technologies Solar Solar Power Generation Radiation fuel Concentrating the solar radiation in Concentrating Absorbing Storage Generation high magnification and using this thermal energy for power generation Absorbing/ fuel Reaction Features of Each Types of Solar Power PTC Type CRS Type Dish type 1Axis Sun tracking controller 2 Axis Sun tracking controller 2 Axis Sun tracking controller Concentrating rate : 30 ~ 100, ~400 oC Concentrating rate: 500 ~ 1,000, Concentrating rate: 1,000 ~ 10,000 ~1,500 oC Parabolic Trough Concentrator Parabolic Dish Concentrator Central Receiver System CSP Technologies PTC CRS Dish commercialized in large scale various types (from 1 to 20MW ) Stirling type in ~25kW size (more than 50MW ) developing the technology, partially completing the development technology development is already commercialized efficiency ~30% reached proper level, diffusion level efficiency ~16% efficiency ~12% CSP Test Facilities Worldwide Parabolic Trough Concentrator In 1994, the first research on high temperature solar technology started PTC technology for steam generation and solar detoxification Parabolic reflector and solar tracking system were developed <The First PTC System Installed in KIER(left) and Second PTC developed by KIER(right)> Dish Concentrator 1st Prototype: 15 circular mirror facets/ 2.2m focal length/ 11.7㎡ reflection area 2nd Prototype: 8.2m diameter/ 4.8m focal length/ 36㎡ reflection area <The First(left) and Second(right) KIER’s Prototype Dish Concentrator> Dish Concentrator Two demonstration projects for 10kW dish-stirling solar power system Increased reflection area(9m dia. 42㎡) and newly designed mirror facets Running with Solo V161 Stirling engine, 19.2% efficiency (solar to electricity) <KIER’s 10kW Dish-Stirling System in Jinhae City> Dish Concentrator 25 20 15 (%) 10 발전 효율 5 Peak. -
Energies for the 21St Century
THE collEcTion 1 w The atom 2 w Radioactivity 3 w Radiation and man 4 w Energy 5 w Nuclear energy: fusion and fission 6 w How a nuclear reactor works 7 w The nuclear fuel cycle 8 w Microelectronics 9 w The laser: a concentrate of light 10 w Medical imaging 11 w Nuclear astrophysics 12 w Hydrogen 13 w The Sun 14 w Radioactive waste 15 w The climate 16 w Numerical simulation 17 w Earthquakes 18 w The nanoworld 19 w Energies for the 21st century © French Alternative Energies and Atomic Energy Commission, 2010 Communication Division Head Office 91191 Gif-sur-Yvette cedex - www.cea.fr ISSN 1637-5408. w Low-carbon energies for a sustainable future FROM RESEARCH TO INDUSTRY 19 w energies for the 21st century InnovatIng for nuclear energy DomestIcatIng solar power BIofuel proDuctIon DevelopIng BatterIes anD fuel cells thermonuclear fusIon 2 w contents century © Jack Star/PhotoLink st Innovating for nuclear ENERgY 6 The beginnings of nuclear energy in France 7 The third generation 8 Generation IV: new concepts 10 DEveloping batteries and fuel cells 25 Domesticating solar Lithium-ion batteries 26 pOwer 13 A different application for Thermal solar power 15 each battery 27 Photovoltaic solar power 16 Hydrogen: an energy carrier 29 Concentrated solar power 19 Thermonuclear fusion 31 BIOFUEL production 20 Tokamak research 33 Biomass 21 ITER project 34 Energies for the 21 2nd generation biofuels 22 Designed and produced by: MAYA press - Printed by: Pure Impression - Cover photo: © Jack Star/PhotoLink - Illustrations : YUVANOE - 09/2010 Low-carbon energies for a sustainable future 19 w Energies for the 21st century w> IntroIntroDuctIon 3 The depletion of fossil resources and global warming are encoura- ging the development of research into new energy technologies (on the left, Zoé, France’s first nuclear reactor, on the right, the national institute for solar power). -
The Economics of Solar Power
The Economics of Solar Power Solar Roundtable Kansas Corporation Commission March 3, 2009 Peter Lorenz President Quanta Renewable Energy Services SOLAR POWER - BREAKTHROUGH OR NICHE OPPORTUNITY? MW capacity additions per year CAGR +82% 2000-08 Percent 5,600-6,000 40 RoW US 40 +43% Japan 10 +35% 2,826 Spain 55 1,744 1,460 1,086 598 Germany 137 241 372 427 2000 01 02 03 04 05 06 07 2008E Demand driven by attractive economics • Strong regulatory support • Increasing power prices • Decreasing solar system prices • Good availability of capital Source: McKinsey demand model; Solarbuzz 1 WE HAVE SEEN SOME INTERESTING CHANGES IN THE U.S. RECENTLY 2 TODAY’S DISCUSSION • Solar technologies and their evolution • Demand growth outlook • Perspectives on solar following the economic crisis 3 TWO KEY SOLAR TECHNOLOGIES EXIST Photovoltaics (PV) Concentrated Solar Power (CSP) Key • Uses light-absorbing material to • Uses mirrors to generate steam characteristics generate current which powers turbine • High modularity (1 kW - 50 MW) • Low modularity (20 - 300 MW) • Uses direct and indirect sunlight – • Only uses direct sunlight – specific suitable for almost all locations site requirements • Incentives widely available • Incentives limited to few countries • Mainly used as distributed power, • Central power only limited by some incentives encourage large adequate locations and solar farms transmission access ~ 10 Global capacity ~ 0.5 GW, 2007 Source: McKinsey analysis; EPIA; MarketBuzz 4 THESE HAVE SEVERAL SUB-TECHNOLOGIES Key technologies Sub technologiesDescription -
FACTBOOK Concentrating Solar Power SBC Energy
LEADING THE ENERGY TRANSITION FACTBOOK Concentrating Solar Power SBC Energy Institute June 2013 © 2013 SBC Energy Institute. All Rights Reserved. FACTBOOK SERIES LEADING THE ENERGY TRANSITION Compiled by the SBC Energy Institute About SBC Energy Institute The SBC Energy Institute, a non-profit organization founded in 2011 at the initiative of Schlumberger Business Consulting (SBC), is a center of excellence for scientific and technological research into issues pertaining to the energy industry in the 21st century. Through its unique capability to leverage both Schlumberger’s technological expertise and SBC’s global network of energy leaders, the SBC Energy Institute is at the forefront of the search for solutions to today’s energy supply challenges. It is overseen by a scientific committee comprised of highly experienced individuals in the areas of natural and applied sciences, business, and petroleum engineering. About Leading the Energy Transition series “Leading the energy transition” is a series of publicly available studies on low-carbon energy technologies conducted by the SBC Energy Institute that aim to provide a comprehensive overview of their development status through a technological and scientific prism. About the Concentrating Solar Power factbook This factbook seeks to capture the current status and future developments of Concentrating Solar Power, detail the main technological hurdles and the areas for Research and Development, and finally analyze the economics of this technology. This factbook has been reviewed by Prof. Dr.-Ing. Robert Pitz-Paal, Co-Director of the Institute of Solar Research from the German Aerospace Center (DLR) and his team. For further information about SBC Energy Institute and to download the report, please visit http://www.sbc.slb.com/sbcinstitute.aspx, or contact us at [email protected] 1 © 2013 SBC Energy Institute. -
The Status of CSP Development
The Status of CSP Development DISH STIRLING POWER TOWER CLFR Tom Mancini CSP Program Manager Sandia National Laboratories PARABOLIC TROUGH 505.844.8643 DISH STIRLING [email protected] [email protected] 1 Presentation Content • Brief Overview of Sandia National Laboratories • Background information • Examples of CSP Technologies − Parabolic Trough Systems − Power Tower Systems − Thermal Energy Storage − Dish Stirling Systems • Status of CSP Technologies • Cost of CSP and Resource Availability • Deployments • R & D Directions [email protected] 2 Four Mission Areas Sandia’s missions meet national needs in four key areas: • Nuclear Weapons • Defense Systems and Assessments • Energy, Climate and Infrastructure Security • International, Homeland, and Nuclear Security [email protected] 3 Research Drives Capabilities High Performance Nanotechnologies Extreme Computing & Microsystems Environments Computer Materials Engineering Micro Bioscience Pulsed Power Science Sciences Electronics Research Disciplines 4 People and Budget . On-site workforce: 11,677 FY10 operating revenue . Regular employees: 8,607 $2.3 billion 13% . Over 1,500 PhDs and 2,500 MS/MA 13% 43% 31% Technical staff (4,277) by discipline: (Operating Budget) Nuclear Weapons Defense Systems & Assessments Energy, Climate, & Infrastructure Security International, Homeland, and Nuclear Security Computing 16% Math 2% Chemistry 6% Physics 6% Other science 6% Other fields 12% Electrical engineering 21% Mechanical engineering 16% Other engineering 15% 5 Sandia’s NSTTF Dish Engine Engine Test Rotating Testing Facility Platform Established in 1976, we provide ………. • CSP R&D NSTTF • Systems analysis and FMEA • System and Tower Testing Solar Furnace component testing and support NATIONAL SOLAR THERMAL TEST FACILITY [email protected] 6 Labs Support the DOE Program The CSP Programs at Sandia and the National Renewable Energy Laboratory (NREL) support the DOE Solar Energy Technology Program. -
Solar Thermal and Concentrated Solar Power Barometers 1 – EUROBSERV’ER –JUIN 2017 – EUROBSERV’ER BAROMETERS POWER SOLAR CONCENTRATED and THERMAL SOLAR
1 2 - 4.6% The decrease of the solar thermal market in the European Union in 2016 Evacuated tube solar collectors, solar thermal installation in Ireland SOLAR THERMAL AND CONCENTRATED SOLAR POWER BAROMETERS A study carried out by EurObserv’ER. solar solar concentrated and thermal power barometers solar solar concentrated and thermal power barometers he European solar thermal market is still losing pace. According to the Tpreliminary estimates from EurObserv’ER, the solar thermal segment dedicated to heat production (domestic hot water, heating and heating networks) contracted by a further 4.6% in 2016 down to 2.6 million m2. The sector is pinning its hopes on the development of the collective solar segment that includes industrial solar heat and solar district heating to offset the under-performing individual home segment. ince 2014 European concentrated solar power capacity for producing Selectricity has been more or less stable. New project constructions have been a long time coming, but this could change at the end of 2017 and in 2018 essentially in Italy. 51 millions m2 2 313.7 MWth The cumulated surfaces of solar thermal Total CSP capacity in operation Glenergy Solar in operation in the European Union in 2016 in the European Union in 2016 SOLAR THERMAL AND CONCENTRATED SOLAR POWER BAROMETERS – EUROBSERV’ER – JUIN 2017 SOLAR THERMAL AND CONCENTRATED SOLAR POWER BAROMETERS – EUROBSERV’ER – JUIN 2017 3 4 The world largest solar thermal Tabl. n° 1 district heating solution - Silkeborg, Denmark (in operation end 2016) Main solar thermal markets outside European Union Total cumulative capacity Annual Installed capacity (in MWth) in operation (in MWth) 2015 2016 2015 2016 China 30 500 27 664 309 500 337 164 United States 760 682 17 300 17 982 Turkey 1 500 1 467 13 600 15 067 India 770 894 6 300 7 194 Japan 100 50 2 400 2 450 Rest of the world 6 740 6 797 90 944 97 728 Total world 39 640 36 660 434 700 471 360 Source: EurObserv’ER 2017 new build, because of the construction is now causing great concern, where as a water production. -
Characterisation of Solar Electricity Import Corridors from MENA to Europe
Characterisation of Solar Electricity Import Corridors from MENA to Europe Potential, Infrastructure and Cost Characterisation of Solar Electricity Import Corridors from MENA to Europe Potential, Infrastructure and Cost July 2009 Report prepared in the frame of the EU project ‘Risk of Energy Availability: Common Corridors for Europe Supply Security (REACCESS)’ carried out under the 7th Framework Programme (FP7) of the European Commission (Theme - Energy-2007-9. 1-01: Knowledge tools for energy-related policy making, Grant agreement no.: 212011). Franz Trieb, Marlene O’Sullivan, Thomas Pregger, Christoph Schillings, Wolfram Krewitt German Aerospace Center (DLR), Stuttgart, Germany Institute of Technical Thermodynamics Department Systems Analysis & Technology Assessment Pfaffenwaldring 38-40 D-70569 Stuttgart, Germany Characterisation of Solar Electricity Import Corridors TABLE OF CONTENTS 1 INTRODUCTION...................................................................................................1 2 STATUS OF KNOWLEDGE - RESULTS FROM RECENT STUDIES .................2 3 EXPORT POTENTIALS – RESOURCES AND PRODUCTION.........................19 3.1 SOLAR ENERGY RESOURCES IN POTENTIAL EXPORT COUNTRIES.........19 3.1.1 Solar Energy Resource Assessment .........................................................19 3.1.2 Land Resource Assessment ......................................................................39 3.1.3 Potentials for Solar Electricity Generation in MENA ..................................48 3.1.4 Potentials for Solar Electricity -
Financing the Transition to Renewable Energy in the European Union
Bi-regional economic perspectives EU-LAC Foundation Miguel Vazquez, Michelle Hallack, Gustavo Andreão, Alberto Tomelin, Felipe Botelho, Yannick Perez and Matteo di Castelnuovo. iale Luigi Bocconi Financing the transition to renewable energy in the European Union, Latin America and the Caribbean Financing the transition to renewable energy in European Union, Latin America and Caribbean EU-LAC / Università Commerc EU-LAC FOUNDATION, AUGUST 2018 Große Bleichen 35 20354 Hamburg, Germany www.eulacfoundation.org EDITION: EU-LAC Foundation AUTHORS: Miguel Vazquez, Michelle Hallack, Gustavo Andreão, Alberto Tomelin, Felipe Botelho, Yannick Perez and Matteo di Castelnuovo GRAPHIC DESIGN: Virginia Scardino | https://www.behance.net/virginiascardino PRINT: Scharlau GmbH DOI: 10.12858/0818EN Note: This study was financed by the EU-LAC Foundation. The EU-LAC Foundation is funded by its members, and in particular by the European Union. The contents of this publication are the sole responsibility of the authors and cannot be considered as the point of view of the EU- LAC Foundation, its member states or the European Union. This book was published in 2018. This publication has a copyright, but the text may be used free of charge for the purposes of advocacy, campaigning, education, and research, provided that the source is properly acknowledged. The co- pyright holder requests that all such use be registered with them for impact assessment purposes. For copying in any other circumstances, or for reuse in other publications, or for translation and adaptation, -
Electric Power Engineering
Renewable Energy Resources – an Overview Y. Baghzouz Professor of Electrical Engineering Overview Solar-derived renewables Photovoltaic (PV) Concentrating Power Systems Biomass Ocean Power Wind Power Hydro Power Earth derived renewables Geothermal Electricity production from renewables HYDRO GEO- THERMAL What is driving the fast growth? The growth in renewables over the past decade is driven mainly by the following: Global concern over the environment. Furthermore, fossil fuel resources are being drained. Renewable technologies are becoming more efficient and cost effective. The Renewable Electricity Production Tax Credit, a federal incentive, encourages the installation of renewable energy generation systems. Many countries have Renewable Portfolio Standards (RPS), which require electricity providers to generate or acquire a percentage of power generation from renewable resources. US States with Renewable Energy Portfolio Standards. CA: 33% by 2020 NV: 25% by 2025 (EU: 20% by 2020) Why not produce more renewable energy? Renewable Energy Technologies Are Capital-Intensive: Renewable energy power plants are generally more expensive to build and to operate than coal and natural gas plants. Recently, however, some wind-generating plants have proven to be economically feasible in areas with good wind resources. Renewable Resources Are Often Geographically Remote: The best renewable resources are often available only in remote areas, so building transmission lines to deliver power to large metropolitan areas is expensive. Wind -
Gamesa Corporación Tecnológica, S.A. and Subsidiaries Composing the GAMESA Group
Gamesa Corporación Tecnológica, S.A. and Subsidiaries composing the GAMESA Group Auditors’ Report Consolidated Financial Statements for the year ended 31 December 2011 prepared in accordance with International Financial Reporting Standards Consolidated Directors' Report Translation of a report originally issued in Spanish based on our work performed in accordance with the audit regulations in force in Spain and of consolidated financial statements originally issued in Spanish and prepared in accordance with the regulatory financial reporting framework applicable to the Group (see Notes 2 and 36). In the event of a discrepancy, the Spanish-language version prevails. Auditor’s report on consolidated annual accounts This version of our report is a free translation of the original, which was prepared in Spanish. All possible care has been taken to ensure that the translation is an accurate representation of the original. However, in all matters of interpretation of information, views or opinions, the original language version of our report takes precedence over this translation To the Shareholders of Gamesa Corporación Tecnológica, S.A. We have audited the consolidated annual accounts of Gamesa Corporación Tecnológica, S.A. (Parent Company) and its subsidiaries (the Group), consisting of the consolidated balance sheet at 31 December 2011, the consolidated income statement, the consolidated statement of comprehensive income, the consolidated statement of changes in equity, the consolidated cash flow statement and related notes to the consolidated annual accounts for the year then ended. As explained in Note 2.a, the Directors of the Parent Company are responsible for the preparation of these consolidated annual accounts in accordance with the International Financial Reporting Standards as endorsed by the European Union, and other provisions of the financial reporting framework applicable to the Group.