Ferric Heme As a CO/NO Sensor in the Nuclear Receptor Rev-Erbß by Coupling Gas Binding to Electron Transfer
Total Page:16
File Type:pdf, Size:1020Kb
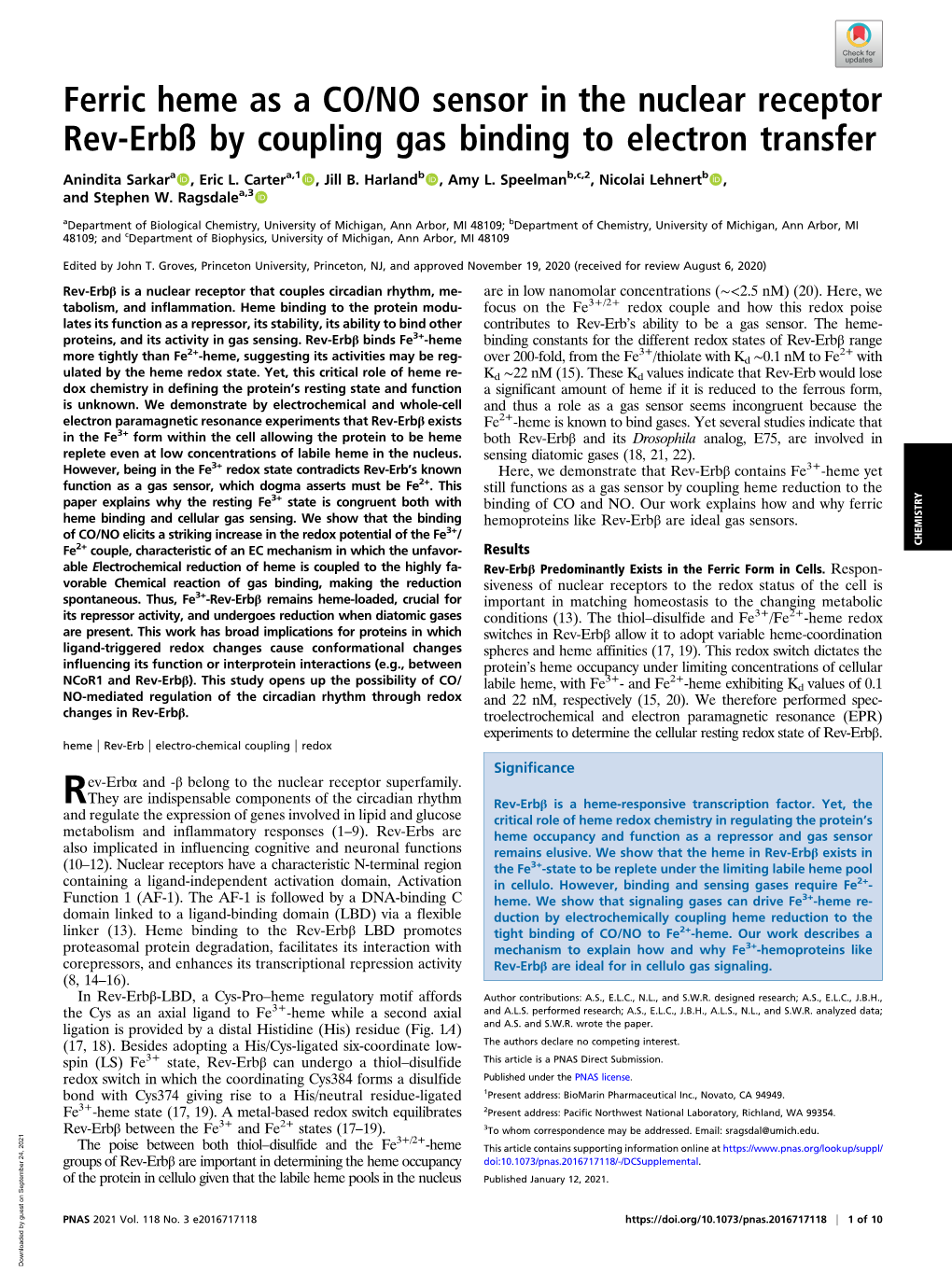
Load more
Recommended publications
-
The Structural Basis of Gas-Responsive Transcription by the Human Nuclear Hormone Receptor REV-Erbb
PLoS BIOLOGY The Structural Basis of Gas-Responsive Transcription by the Human Nuclear Hormone Receptor REV-ERBb Keith I. Pardee1,2, Xiaohui Xu1,2,3, Jeff Reinking1,2,7¤a, Anja Schuetz4¤b, Aiping Dong4, Suya Liu5, Rongguang Zhang6, Jens Tiefenbach1,2, Gilles Lajoie5, Alexander N. Plotnikov4¤b, Alexey Botchkarev4, Henry M. Krause1,2*, Aled Edwards1,2,3,4* 1 Banting and Best Department of Medical Research, The Department of Molecular Genetics, University of Toronto, Toronto, Canada, 2 Terrence Donnelly Centre for Cellular & Biomolecular Research, University of Toronto, Toronto, Canada, 3 Midwest Center for Structural Genomics, University of Toronto, Toronto, Canada, 4 Structural Genomics Consortium, University of Toronto, Toronto, Canada, 5 Department of Biochemistry, University of Western Ontario, London, Ontario, Canada, 6 Midwest Center for Structural Genomics, Argonne National Lab, Argonne, Illinois, United States of America, 7 Department of Biology, State University of New York at New Paltz, New Paltz, New York, United States of America Heme is a ligand for the human nuclear receptors (NR) REV-ERBa and REV-ERBb, which are transcriptional repressors that play important roles in circadian rhythm, lipid and glucose metabolism, and diseases such as diabetes, atherosclerosis, inflammation, and cancer. Here we show that transcription repression mediated by heme-bound REV- ERBs is reversed by the addition of nitric oxide (NO), and that the heme and NO effects are mediated by the C-terminal ligand-binding domain (LBD). A 1.9 A˚ crystal structure of the REV-ERBb LBD, in complex with the oxidized Fe(III) form of heme, shows that heme binds in a prototypical NR ligand-binding pocket, where the heme iron is coordinately bound by histidine 568 and cysteine 384. -
Study of the Role of the Adaptor Protein Myd88 in the Iron-Sensing Pathway
Université de Montréal Study of the role of the adaptor protein MyD88 in the iron-sensing pathway and of the effect of curcumin in the development of anemia in a DSS-induced colitis mouse model By Macha Samba Mondonga Department of Microbiology and Immunology Faculty of Medicine Thesis presented to the Faculty of Graduate Studies in partial fulfillment of the Ph.D. degree in Microbiology, Immunology and Infectious Diseases 30 Aout 2018 © Macha Samba Mondonga, 2018 Université de Montréal Cette thése est intitulée: Study of the role of the adaptor protein MyD88 in the iron-sensing pathway and of the effect of curcumin in the development of anemia in a DSS-induced colitis mouse model Par Macha Samba Mondonga Department of Microbiology and Immunology Faculty of Medicine 30 Aout 2018 © Macha Samba Mondonga, 2018 RÉSUMÉ Étude du rôle de la protéine adaptatrice MyD88 dans la voie de détection du fer et de l’effet de la curcumine dans le développement de l'anémie dans un modèle murin de colite induite par le DSS L'hepcidine est l'hormone peptidique essentielle pour la régulation systémique de l'homéostasie du fer, en déclenchant la dégradation de la ferroportine et en évitant le développement d'une surcharge ou d'une carence en fer. L'expression de l'ARNm de l'hepcidine HAMP est contrôlée par deux voies principales, la voix de signalisation inflammatoire et la voie de détection en fer, BMP/SMAD4. Il a été rapporté que MyD88, la protéine adaptatrice impliquée dans l'activation des cytokines via la stimulation des récepteurs toll-like (TLRs), contribue à l'expression de l'hepcidine dans la voie inflammatoire. -
Data-Driven Insights Into Ligands, Proteins, and Genetic Mutations
Data-Driven Insights into Ligands, Proteins, and Genetic Mutations by Jing Lu A dissertation submitted in partial fulfillment of the requirements for the degree of Doctor of Philosophy (Bioinformatics) in the University of Michigan 2016 Doctoral Committee: Professor Heather A. Carlson, Chair Professor Charles L. Brooks III Assistant Professor Barry Grant Professor David S. Sept Professor Kerby A. Shedden © Jing Lu, 2016 Acknowledgements I would like to thank my advisor, Dr. Heather Carlson, for years of patient guidance, teaching, and support through the course of my PhD. I have learnt how to think critically and be rigorous in every step of research. I also want to express gratitude to my committee: Professor Charles L. Brooks III, Assistant Professor Barry Grant, Professor David S. Sept, Professor Kerby A. Shedden. Their advising is insightful and deepens my understanding of my research projects. I would like to thank Dr. Richard Smith for timely support for both my writing and research. For many Saturdays and Sundays, he promptly responds my requests for proofreading. Much of my work is built on his code in protein and ligand analysis. I would like to thank other members in Dr. Carlson’s lab for helping me with my work. Through the discussion with Dr. Jim Dunbar, I have learnt many critical ideas in Cheminformatics. Also, thank you to Sarah Graham and Jordan Clark for their tremendous friendship and willing to help with my writing. I would also thank previous members in Dr. Carlson’s lab. I would thank Dr. Phani Ghanakota for many late-night discussions and Dr. -
Targeting Heme with Single Domain Antibodies
Targeting Heme with Single Domain Antibodies Zélia Licínia Ferreira Gouveia Dissertation presented to obtain the Ph.D degree in Biochemistry Instituto de Tecnologia Química e Biológica António Xavier | Universidade Nova de Lisboa Oeiras, June, 2015 Targeting Heme with Single Domain Antibodies Zélia Licínia Ferreira Gouveia Dissertation presented to obtain the Ph.D degree in Biochemistry Instituto de Tecnologia Química e Biológica António Xavier | Universidade Nova de Lisboa Research work coordinated by: Oeiras, Junho, 2015 Targeting Heme with Single Domain Antibodies Zélia Licínia Ferreira Gouveia Supervisor: Dr. Miguel P.Soares Instituto Gulbenkian da Ciência Co-Supervisor: Dr. Smilja Todorovic Instituto de Tecnologia Química e Biológica PhD fellowship financed by Fundação para a Ciência e Tecnologia (Apoio financeiro da FCT e do FSE no âmbito do Quadro Comunitário de apoio, BD: SFRH/BD/44828/2008) Oeiras, Junho, 2015 Acknowledgements Firstly and primarily I would like to express my most sincere gratitude to my Ph.D supervisor Dr. Miguel P. Soares for accepting me as his Ph.D student and to trust me this Ph.D project. I want to thank him for his help, advices and for non-stop teaching and extremely helpful and constructive scientific discussions. Today, I am the scientist that you help to build, thank you very much! I extremely grateful to Dr. Miguel P. Soares for his understanding and support, in a particular period of my life in which I had to take several weeks of my work time to be and support my family as we were having a extremely difficult time in our life. For all of this and more, thank you very much! I am grateful to Dr. -
(12) Patent Application Publication (10) Pub. No.: US 2011/0098193 A1 Kingsmore Et Al
US 2011 0098193A1 (19) United States (12) Patent Application Publication (10) Pub. No.: US 2011/0098193 A1 Kingsmore et al. (43) Pub. Date: Apr. 28, 2011 (54) METHODS AND SYSTEMS FOR MEDICAL type (such as a disease symptom) of the trait, wherein the SEQUENCING ANALYSIS association of the relevant element with the relevant compo nent phenotype identifies the relevant element as an element (76) Inventors: Stephen F. Kingsmore, St. associated with the trait, wherein the relevant component Augustine, FL (US); Callum J. phenotype is a component phenotype having a threshold Bell, Santa Fe, NM (US) value of severity, age of onset, specificity to the trait or dis ease, or a combination, wherein the relevant element is an (21) Appl. No.: 12/910,764 element having a threshold value of importance of the ele ment to homeostasis relevant to the trait, intensity of the (22) Filed: Oct. 22, 2010 perturbation of the element, duration of the effect of the element, or a combination. The disclosed methods are based Related U.S. Application Data on a model of how elements affect complex diseases. The disclosed model is based on the existence of significant (60) Provisional application No. 61/254,115, filed on Oct. genetic and environmental heterogeneity in complex dis 22, 2009. eases. Thus, the specific combinations of genetic and envi ronmental elements that cause disease vary widely among the Publication Classification affected individuals in a cohort. The disclosed model is an (51) Int. Cl. effective, general experimental design and analysis approach C4OB 30/04 (2006.01) for the identification of causal variants in common, complex C4DB 60/2 (2006.01) diseases by medical sequencing. -
Mechanisms of Paracrine and Endocrine Actions of Angiotensin II
University of Tennessee, Knoxville TRACE: Tennessee Research and Creative Exchange Doctoral Dissertations Graduate School 8-2004 Mechanisms of paracrine and endocrine actions of Angiotensin II Suyeon Kim University of Tennessee, Knoxville Follow this and additional works at: https://trace.tennessee.edu/utk_graddiss Part of the Life Sciences Commons Recommended Citation Kim, Suyeon, "Mechanisms of paracrine and endocrine actions of Angiotensin II. " PhD diss., University of Tennessee, 2004. https://trace.tennessee.edu/utk_graddiss/4533 This Dissertation is brought to you for free and open access by the Graduate School at TRACE: Tennessee Research and Creative Exchange. It has been accepted for inclusion in Doctoral Dissertations by an authorized administrator of TRACE: Tennessee Research and Creative Exchange. For more information, please contact [email protected]. To the Graduate Council: I am submitting herewith a dissertation written by Suyeon Kim entitled "Mechanisms of paracrine and endocrine actions of Angiotensin II." I have examined the final electronic copy of this dissertation for form and content and recommend that it be accepted in partial fulfillment of the requirements for the degree of Doctor of Philosophy, with a major in Human Ecology. Naima Moustaid-Moussa, Major Professor We have read this dissertation and recommend its acceptance: Jay Whelan, Jung Han Kim, Brynn H. Jones, John Koontz Accepted for the Council: Carolyn R. Hodges Vice Provost and Dean of the Graduate School (Original signatures are on file with official studentecor r ds.) To the Graduate Council: I am submitting herewith a dissertationwritten by Suyeon Kim entitled "Mechanisms of paracrine and endocrineactions of Angiotensin II." I have examined the finalpaper copy of this dissertation for formand content and recommend that it be accepted in partial fulfillment of the requirements forthe degreeof Doctor of Philosophy, with a major in Human Ecology. -
The Incidence and Functional Relevance of Intrinsic Disorder in Enzymes and the Protein Data Bank Shelly Deforte University of South Florida, [email protected]
University of South Florida Scholar Commons Graduate Theses and Dissertations Graduate School 6-27-2016 Intrinsic Disorder Where You Least Expect It: The Incidence and Functional Relevance of Intrinsic Disorder in Enzymes and the Protein Data Bank Shelly Deforte University of South Florida, [email protected] Follow this and additional works at: http://scholarcommons.usf.edu/etd Part of the Bioinformatics Commons, Medicine and Health Sciences Commons, and the Molecular Biology Commons Scholar Commons Citation Deforte, Shelly, "Intrinsic Disorder Where You Least Expect It: The ncI idence and Functional Relevance of Intrinsic Disorder in Enzymes and the Protein Data Bank" (2016). Graduate Theses and Dissertations. http://scholarcommons.usf.edu/etd/6219 This Thesis is brought to you for free and open access by the Graduate School at Scholar Commons. It has been accepted for inclusion in Graduate Theses and Dissertations by an authorized administrator of Scholar Commons. For more information, please contact [email protected]. Intrinsic Disorder Where You Least Expect It: The Incidence and Functional Relevance of Intrinsic Disorder in Enzymes and the Protein Data Bank by Shelly DeForte A dissertation submitted in partial fulfillment of the requirements for the degree of Doctor of Philosophy Department of Molecular Medicine College of Medicine University of South Florida Major Professor: Vladimir Uversky, Ph.D. Yu Chen, Ph.D. Robert Deschenes, Ph.D. Sandy Westerheide, Ph.D. Bin Xue, Ph.D. Date of Approval: June 14, 2016 Keywords: intrinsically disordered protein, x-ray crystallography, structural biology, enzyme function Copyright © 2016, Shelly DeForte Table of Contents List of Tables ................................................................................................ iv List of Figures ............................................................................................... -
Acronyms/Abbreviations
NOAA Coral Reef Information System - Glossary of Terminology Coral Reef Information System Home Data & Publications Regional Portals CRCP Activities Glossary Home / Glossary Home / Glossary of Terminology and Acronyms/Abbreviations Acronyms/Abbreviations A | B | C | D | E | F | G | H | I | J | K | L | M | N | O | P | Q | R | S | T | U | V | W | X | Y | Z | 20CR : 20th Century Reanalysis A | B | C | D | E | F | G | H | I | J | K | L | M | N | O | P | Q | R | S | T | U | V | W | X | Y | Z | A : Adenine A/D : Analog/Digital A3H : Activities and Attractions Association of Hawaii AA : Alien Algae AA : Assistant Administrator AA : Atomic Absorption AA : Awareness and Appreciation AA : Awareness and Appreciation Team (SEFCRI) AAAS : American Association for the Advancement of Science AACL : Aquaculture, Aquarium, Conservation and Legislation http://coralglossaryxml.s3-website-us-west-2.amazonaws.com/print-acronym.html[9/13/2015 3:46:07 PM] NOAA Coral Reef Information System - Glossary of Terminology AAFC : Agriculture and Agri-Food Canada AAG : American Association of Geographers AAL : Average Annual Loss AAPA : American Association of Port Authorities AAPG : American Association of Petroleum Geologists AAR : Amino Acid Racemization AARNet : Australian Academic and Research Network AAS : Alternate Air Source AAS : Atomic Absorption Spectrometry AATAMS : Australian Acoustic Tagging and Monitoring System AAUS : American Academy of Underwater Sciences ABC : Acceptable Biological Catch ABC : ATP Binding Cassette ABE : Autonomous Benthic Explorer -
Ferric Heme As a CO/NO Sensor in the Nuclear Receptor Reverbβ by Coupling 2 Gas Binding to Electron Transfer 3 Anindita Sarkar1, Eric L
bioRxiv preprint doi: https://doi.org/10.1101/2020.06.22.164806; this version posted June 22, 2020. The copyright holder for this preprint (which was not certified by peer review) is the author/funder, who has granted bioRxiv a license to display the preprint in perpetuity. It is made available under aCC-BY-NC-ND 4.0 International license. 1 Ferric Heme as a CO/NO Sensor in the Nuclear Receptor Reverbβ by Coupling 2 Gas binding to Electron Transfer 3 Anindita Sarkar1, Eric L. Carter1, Jill B. Harland2, Amy L. Speelman2,3, Nicolai Lehnert2, and Stephen W. 4 Ragsdale1* 5 1Department oF Biological Chemistry, University oF Michigan, Ann Arbor, Michigan 48109. 6 2Department oF Chemistry and Department of Biophysics, University of Michigan, Ann Arbor, Michigan 48109. 7 3Current address: PaciFic Northwest National Laboratory, Richland, WA 99354. 8 9 Abstract 10 Rev-Erbβ is a nuclear receptor that couples circadian rhythm, metabolism, and inflammation.1-7 11 Heme binding to the protein modulates its function as a repressor, its stability, its ability to bind 12 other proteins, and its activity in gas sensing.8-11 Rev-Erbβ binds Fe3+-heme tighter than Fe2+- 13 heme, suggesting its activities may be regulated by the heme redox state.9 Yet, this critical role of 14 heme redox chemistry in defining the protein’s resting state and function is unknown. We 15 demonstrate by electrochemical and whole-cell electron paramagnetic resonance experiments that 16 Rev-Erbβ exists in the Fe3+ form within the cell essentially allowing the protein to be heme-replete 17 even at low concentrations of labile heme in the nucleus. -
Biology Is the Most Powerful Technology on the Planet
biology is the most powerful technology on the planet WE’RE HIRING ginkgobioworks.com/careers One source. Multiple solutions. Wherever your research takes you, whatever the latest technology, you can rely on our real-industry expertise and high quality, trusted products. Together, we can create long-lasting partnerships that stand the test of time and technology. See what we can do for you at www.idtdna.com. table of contents About ......................................................................................................... 9 Team Map ................................................................................................... 10 Contributors ............................................................................................... 12 Sponsors ..................................................................................................... 18 Exhibitors ................................................................................................... 19 Maps ......................................................................................................... 20 Exterior Hynes Convention Center...................................................... 20 Plaza Level ....................................................................................... 21 Halls A and B ..................................................................................... 22 Second Floor..................................................................................... 23 Third Floor....................................................................................... -
ROLE of BIOACTIVE FOOD COMPOUNDS in ACUTE and CHRONIC INFLAMMATION Victor Pallarés López
ROLE OF BIOACTIVE FOOD COMPOUNDS IN ACUTE AND CHRONIC INFLAMMATION Victor Pallarés López Dipòsit Legal: T.1472-2012 ADVERTIMENT. L'accés als continguts d'aquesta tesi doctoral i la seva utilització ha de respectar els drets de la persona autora. Pot ser utilitzada per a consulta o estudi personal, així com en activitats o materials d'investigació i docència en els termes establerts a l'art. 32 del Text Refós de la Llei de Propietat Intel·lectual (RDL 1/1996). Per altres utilitzacions es requereix l'autorització prèvia i expressa de la persona autora. En qualsevol cas, en la utilització dels seus continguts caldrà indicar de forma clara el nom i cognoms de la persona autora i el títol de la tesi doctoral. No s'autoritza la seva reproducció o altres formes d'explotació efectuades amb finalitats de lucre ni la seva comunicació pública des d'un lloc aliè al servei TDX. Tampoc s'autoritza la presentació del seu contingut en una finestra o marc aliè a TDX (framing). Aquesta reserva de drets afecta tant als continguts de la tesi com als seus resums i índexs. ADVERTENCIA. El acceso a los contenidos de esta tesis doctoral y su utilización debe respetar los derechos de la persona autora. Puede ser utilizada para consulta o estudio personal, así como en actividades o materiales de investigación y docencia en los términos establecidos en el art. 32 del Texto Refundido de la Ley de Propiedad Intelectual (RDL 1/1996). Para otros usos se requiere la autorización previa y expresa de la persona autora. En cualquier caso, en la utilización de sus contenidos se deberá indicar de forma clara el nombre y apellidos de la persona autora y el título de la tesis doctoral. -
M. Muoio and Daniel P. Kelly Kapoor, Timothy R. Koves, Robert Stevens
Energy Metabolic Re-Programming in the Hypertrophied and Early Stage Failing Heart: A Multi-systems Approach Ling Lai, Teresa C. Leone, Mark P. Keller, Ola J. Martin, Aimee T. Broman, Jessica Nigro, Kapil Kapoor, Timothy R. Koves, Robert Stevens, Olga R. Ilkayeva, Rick B. Vega, Alan D. Attie, Deborah M. Muoio and Daniel P. Kelly Circ Heart Fail. published online September 18, 2014; Circulation: Heart Failure is published by the American Heart Association, 7272 Greenville Avenue, Dallas, TX 75231 Copyright © 2014 American Heart Association, Inc. All rights reserved. Print ISSN: 1941-3289. Online ISSN: 1941-3297 The online version of this article, along with updated information and services, is located on the World Wide Web at: http://circheartfailure.ahajournals.org/content/early/2014/09/18/CIRCHEARTFAILURE.114.001469 Data Supplement (unedited) at: http://circheartfailure.ahajournals.org/content/suppl/2014/09/18/CIRCHEARTFAILURE.114.001469.DC1.html Permissions: Requests for permissions to reproduce figures, tables, or portions of articles originally published in Circulation: Heart Failure can be obtained via RightsLink, a service of the Copyright Clearance Center, not the Editorial Office. Once the online version of the published article for which permission is being requested is located, click Request Permissions in the middle column of the Web page under Services. Further information about this process is available in the Permissions and Rights Question and Answer document. Reprints: Information about reprints can be found online at: http://www.lww.com/reprints Subscriptions: Information about subscribing to Circulation: Heart Failure is online at: http://circheartfailure.ahajournals.org//subscriptions/ Downloaded from http://circheartfailure.ahajournals.org/ at Loyola University of Chicago on November 3, 2014 Energy Metabolic Re-Programming in the Hypertrophied and Early Stage Failing Heart: A Multi-systems Approach Lai et al: Metabolic Remodeling in Heart Failure Ling Lai, MD, PhD1; Teresa C.