Inspiration from Design and Assembly
Total Page:16
File Type:pdf, Size:1020Kb
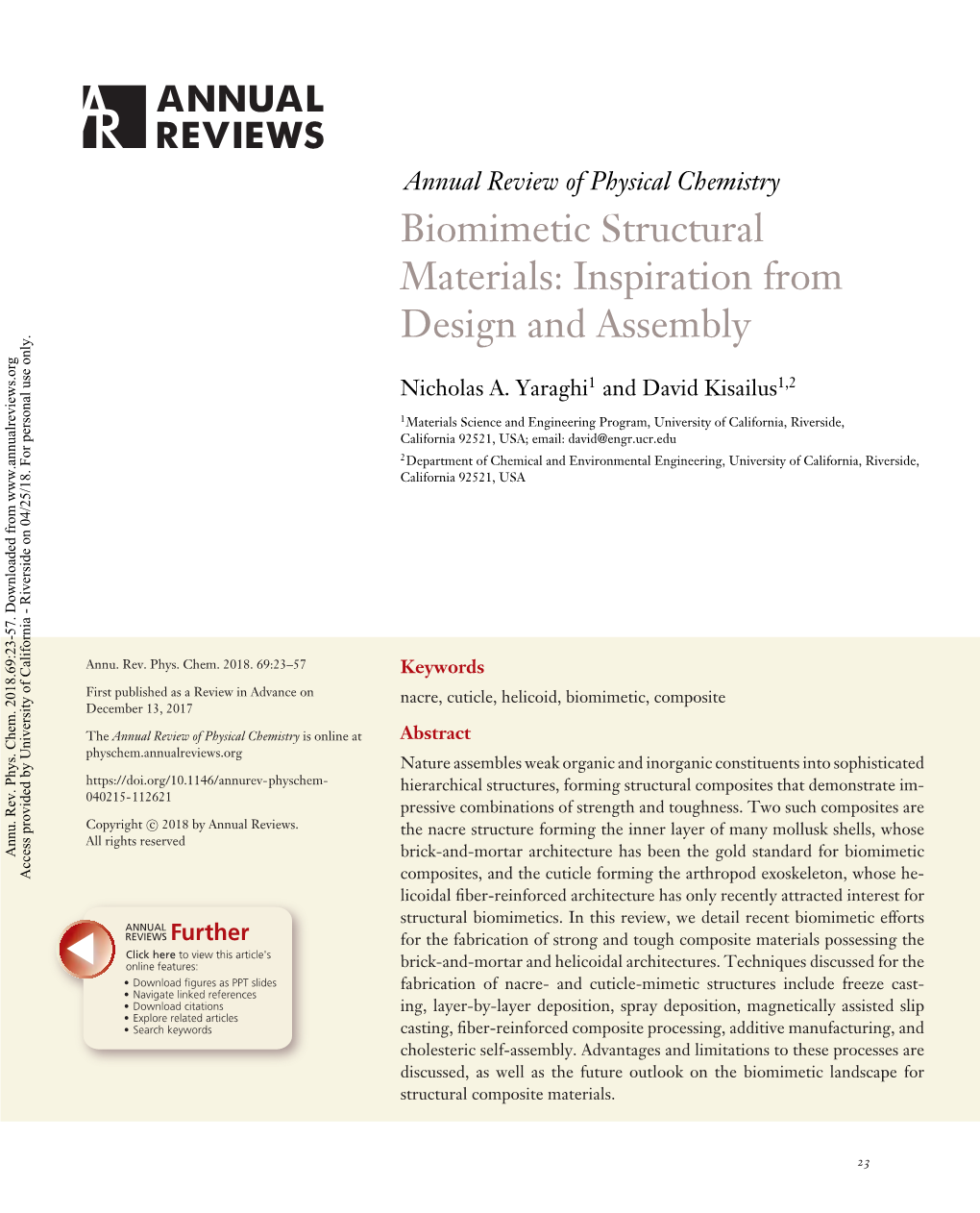
Load more
Recommended publications
-
Chapter 14 1. Specialization of Regions of the Body for Specific
Chapter 14 1. Specialization of regions of the body for specific functions, as seen in arthropods, is called A) tagmatization. B) metamerism. C) truncation. D) differentiation. E) cephalization. 2. Members of class __________ are among the most numerous crustaceans, and are both marine and freshwater in distribution. A) Cirripedia B) Copepoda C) Branchiopoda D) Malacostraca E) Isopoda 3. Which type of crustacean do many zoologists believe to have the greatest number of individuals of any type of animal on the planet? A) isopods B) fairy shrimp C) brine shrimp D) copepods E) barnacles 4. Which of the following phyla of animals are not ecdysozoan? A) Arthropoda B) Nematoda C) Gastrotricha D) Nematomorpha E) Kinorhyncha 5. Which of the following is not a synapomorphy that unites the members of the ecdysozoan clade? A) the blastopore develops into the anus B) loss of epidermal cilia C) possession of a cuticle D) shedding of cuticle through ecdysis E) all of the above are synapomorphies shared by ecdysozoans Page 1 6. The __________ is the outer layer of the arthropod exoskeleton, and it is composed of a waterproofing waxy lipoprotein. A) lipocuticle B) mesocuticle C) epicuticle D) endocuticle E) sclerocuticle 7. The tough, leathery polysaccharide in the arthropod procuticle is A) lipoprotein. B) calcium carbonate. C) scleroprotein. D) chitin. E) glycogen. 8. The arthropod skeleton hardens by __________, which is a formation of chemical bonds between protein chains. A) carbonization B) tagmatization C) calcification D) chitinization E) sclerotization 9. Sensory receptors called __________ occur in the arthropod exoskeleton in the form of pegs, bristles, and lenses. -
Research Interests Related to the Cambridge-MIT Institute
Nanoscale Structural Design Principles of Biocomposite Exoskeletons As a Guide for New Energy-Absorbing Materials Technologies Team 1 : Energy Absorbing Materials : Multiscale Design and Evaluation of Nanostructured Materials for Ballistic and Blast Protection MIT Institute for Soldier Technologies (ISN) Christine Ortiz, Assistant Professor Massachusetts Institute of Technology, Department of Materials Science and Engineering WWW : http://web.mit.edu/cortiz/www/ August 2002 I. Background : Structural Design Principles and Energy Absorbing Mechanisms From millions of years of evolution, nature has ingeniously figured out innumerate structural design principles to produce multifunctional, and in many cases stimulus-responsive, materials with superior mechanical properties[1-6]. Examples of these include exoskeletons of many invertebrate animals such as mollusks, arthropods (e.g. crustaceans such as crabs, insects), cnidaria (e.g. corals), and structural components of mammals such as turtle shell, rhinocerous horn, bovine hoof horn, deer antlers, elephant tusks, and teeth. Most tough biological materials are complex, hierarchical, multilayered nanocomposites that undergo a wide variety of different energy-absorbing toughening mechanisms at many length scales. Some of these mechanisms in both biological and synthetic composite materials [7-10] are shown in Figure 1 and include; 1) rupture of "sacrificial" weaker bonds in the macromolecular component (e.g. Mollusk shell nacre), 2) extension, pull-out, and/or ligament formation of a macromolecular component bridging an interface (e.g. Mollusk shell nacre), 3) void formation (e.g. via cavitation of rubber particles in a thermoset composite or stress whitening in semicrystalline polymers) leading to bulk plastic deformation, crack blunting, pinning and branching, 4) localized plastic deformation ahead of a crack tip (e.g. -
CH33 Arthropods All.Pptx
10/15/14 Biosc 41 Announcements 10/15 Quick Platyhelminthes Review v What type of symmetry do animals in Phylum v Quiz today- Phyla Platyhelminthes and Mollusca Platyhelminthes have? v v Lecture- Phylum Arthropoda Digestive system: mouth only (protostomes) v How does gas exchange take place? v Lab- Phylum Arthropoda v What are protonephridia for? v What is the class of free-living flatworms? An v Mon’s lecture will finish any arthropod info we don’t get to example? today, have arthropod quiz, and do an exam review activity v What are the classes of parasitic flatworms? Example? v Mon’s lab will finish arthropod lab material (+ crayfish dissection) Quick Mollusca Review Quick Mollusca Review v Features and structures universal amongst molluscs: v Four major classes: § Mantle- secretes shell in shelled molluscs; sometimes § Class Polyplacophora- Chitons portions of mantle form siphon(s) § Class Gastropoda- snails, nudibranchs, slugs § Foot- contains sensory cells; adapted in various ways in § Life cycle includes trochophore & veliger larvae different classes § Veliger stage involves torsion § Visceral mass- internal organs consistent between classes, but arranged in different orientations § Class Bivalvia- clams, oysters, mussels § Life cycle includes trochophore & veliger larvae § Circulatory system includes heart and is open in all but cephalopods (which have a closed circulatory system) § Class Cephalopoda- squid, octopus, cuttlefish, nautilus § Nervous system includes nerve cords and centralized brain tissue § Mantle has been modified to include a siphon for locomotion § Digestive system includes radula, well-developed digestive gland, and “complete gut” § Foot has been modified into tentacles § Heart and kidneys function as excretory system § Closed circulation and complex brain Quick Mollusca Review Quiz 5 v What is a possible adverse effect of suspension feeding? 1. -
The Crusty Fossils
Curriculum Units by Fellows of the Yale-New Haven Teachers Institute 1985 Volume VII: Skeletal Materials- Biomineralization The Crusty Fossils Curriculum Unit 85.07.03 by Carolyn C. Smith Stop! Look! Listen! These three basic commands can be associated with any adventure that a person wishes to undertake. As this unit is being presented those three little words will play a significant role. The changes which occur on this earth are so gradual that we don’t notice them immediately. However, if we were to ignore our surroundings for a few days or weeks, we would be able to recognize a difference of some kind almost instantly. When we finally notice the changes we are quick to say, “It happened overnight!” Scientists are constantly recording the changes which are occurring on this earth. For centuries man has theorized why, when, where, who, what, and how something is done. Ever so often new evidence is presented and he is forced to change his explanations when results contradict his previous understanding. This unit deals with the results of a chain of events which is believed to have started 4-1/2 billion years ago. We are going to take a look at a species of animals which is found in the Arthropoda Kingdom—“The Crab”. You will find that these little creatures are quite fascinating in their developmental and behavior patterns. After studying this unit the students will be able to: 1. identify the Phylum Arthropoda. 2. discuss the Class Crustacea. 3. discuss the evolution of the crabs. 4. discuss the stages of development of crabs. -
A Closer Look at Arthropods 701 DO NOT EDIT--Changes Must Be Made Through “File Info” Correctionkey=A
DO NOT EDIT--Changes must be made through “File info” CorrectionKey=A A Closer Look CHAPTER 24 at Arthropods BIg IdEa Arthropods are invertebrates and are the most abundant and diverse of all animals. 24.1 Arthropod diversity 7A, 7E, 8C 24.2 Crustaceans 7A, 7E, 8C 24.3 Arachnids 8C data analysis Constructing SCatterplots 2F, 2G 24.4 Insect adaptations 24.5 Arthropods and Humans Online BiOlOgy HMDScience.com ONLINE Labs ■■ Virtual Lab Insects and Crime Scene Analysis ■■ QuickLab Comparing Arthropods ■■ Video Lab Butterfly Metamorphosis ■■ Hatching Brine Shrimp ■■ S.T.E.M. Lab Exoskeleton Strength ■■ Daphnia and Heart Rate ■■ Inside a Crayfish ■■ Identifying Arthropods in a Decomposer System ■■ Determining Time of Death Using Entomology (t) Tenaglia-missouriplants.com ©Dan 700 Unit 8: Animals DO NOT EDIT--Changes must be made through “File info” CorrectionKey=A Q What is the relationship between these two insects? Arthropod predators such as this digger wasp help to keep an important balance among Earth’s invertebrates. This digger wasp has captured a meal, not for itself but for its young. The wasp will deposit the live, but paralyzed, grasshopper into a burrow she has constructed. She will then lay a single egg next to the grasshopper so when the egg hatches the larva will have a fresh meal. r E a d IN g T o o lb o x This reading tool can help you learn the material in the following pages. uSINg LaNGUAGE YOur TurN Classification Categories are groups of things that Read the following sentences. Identify the category and have certain characteristics in common. -
Ultrastructure, Histochemistry, and Mineralization Patterns in the Ecdysial Suture of the Blue Crab, Callinectes Sapidus
ULTRASTRUCTURE, HISTOCHEMISTRY, AND MINERALIZATION PATTERNS IN THE ECDYSIAL SUTURE OF THE BLUE CRAB, CALLINECTES SAPIDUS Carolina Priester A Thesis Submitted to the University of North Carolina at Wilmington in Partial Fulfillment of the Requirements for the Degree of Master of Science Department of Biological Sciences University of North Carolina at Wilmington 2003 Approved by Advisory Committee Neil Hadley Robert Roer Dr. Neil Hadley Dr. Robert Roer Thomas H. Shafer Richard M. Dillaman Dr. Thomas Shafer Dr. Richard Dillaman, Chair Accepted by Robert Roer Dean, Graduate School This thesis has been prepared in the style and format consistent with the journal Journal of Morphology ii TABLE OF CONTENTS ABSTRACT....................................................................................................................... iv ACKNOWLEDGEMENTS............................................................................................... vi DEDICATION.................................................................................................................. vii LIST OF TABLES........................................................................................................... viii LIST OF FIGURES ........................................................................................................... ix INTRODUCTION ...............................................................................................................1 MATERIALS AND METHODS.........................................................................................5 -
Preferred Crystallographic Texture of A-Chitin As a Microscopic and Macroscopic Design Principle of the Exoskeleton of the Lobster Homarus Americanus
Acta Biomaterialia 3 (2007) 882–895 www.elsevier.com/locate/actabiomat Preferred crystallographic texture of a-chitin as a microscopic and macroscopic design principle of the exoskeleton of the lobster Homarus americanus D. Raabe a,*, A. Al-Sawalmih b, S.B. Yi c, H. Fabritius a a Max-Planck-Institut fu¨r Eisenforschung, Max-Planck-Street 1, D-40237 Du¨sseldorf, Germany b Max-Planck-Institute of Colloids and Interfaces, Wissenschaftspark Golm, D-14424 Potsdam, Germany c Institut fu¨r Werkstoffkunde und Werkstofftechnik, TU-Clausthal, Agricolastr. 6, D-38678 Clausthal-Zellerfeld, Germany Received 5 February 2007; received in revised form 4 April 2007; accepted 16 April 2007 Available online 14 June 2007 Abstract The crystallographic texture of the crystalline a-chitin matrix in the biological composite material forming the exoskeleton of the lob- ster Homarus americanus has been determined using synchrotron X-ray pole figure measurements and the calculation of orientation dis- tribution functions. The study has two objectives. The first one is to elucidate crystallographic building principles via the preferred synthesis of certain orientations in crystalline organic tissue. The second one is to study whether a general global design principle exists for the exoskeleton which uses preferred textures relative to the local coordinate system throughout the lobster cuticle. The first point, hence, pursues the question of the extent to which and why a-chitin reveals preferred textures in the lobster cuticle. The second point addresses the question of why and whether such preferred textures (and the resulting anisotropy) exist everywhere in the exoskeleton. Concerning the first aspect, a strong preference of a fiber texture of the orthorhombic a-chitin is observed which is characterized by a h020i crystal axis normal to the exoskeleton surface for the chitin matrix. -
How and Why Did the Arthropod Shed Its Skin? Moulting in Living and Fossil Arthropods Author(S): Harriet B
Title: Patterns in palaeontology: How and why did the arthropod shed its skin? Moulting in living and fossil arthropods Author(s): Harriet B. Drage* 1 Volume: 6 Article: 7 Page(s): 110. Published Date: 01/07/2016 PermaLink: http://www.palaeontologyonline.com/articles/2016/arthropodmoulting/ IMPORTANT Your use of the Palaeontology [online] archive indicates your acceptance of Palaeontology [online]'s Terms and Conditions of Use, available at h ttp://www.palaeontologyonline.com/siteinformation/termsandconditions/. COPYRIGHT Palaeontology [online] (www.palaeontologyonline.com) publishes all work, unless otherwise stated, under the Creative Commons Attribution 3.0 Unported (CC BY 3.0) license. This license lets others distribute, remix, tweak, and build upon the published work, even commercially, as long as they credit Palaeontology[online] for the original creation. This is the most accommodating of licenses offered by Creative Commons and is recommended for maximum dissemination of published material. Further details are available at http://www.palaeontologyonline.com/siteinformation/copyright/. CITATION OF ARTICLE Please cite the following published work as: Drage, Harriet B. 2016. Patterns in palaeontology: How and why did the arthropod shed its skin? Moulting in living and fossil arthropods, Palaeontology Online, Volume 6, Article 7, 110. Patterns in palaeontology: How and why did the arthropod shed its skin? Moulting in living and fossil arthropods by H arriet B. Drage* 1 Introduction: Arthropods are one of the most successful groups of animals, in the present day and the fossil record. There are more than 1 million described arthropod species, and it has been estimated that there are at least 5 million more undescribed alive today (Fig. -
The Arthropod Cuticle 8 Molting
An Introduction to the Invertebrates, Arthropods, Part One Reference: Chapter 33 "Don't accept the chauvinistic tradition that labels our era the age of mammals. This is the age of arthropods. They outnumber us by any criterion – by species, by individuals, by prospects for evolutionary continuation." Stephen Jay Gould, 1988 More Relationships… Arthropod = “jointed leg” v Eumetazoan protostome, bilateral symmetry, triploblastic, coelomate (mostly) v Defining characteristics § Segmented body § Some segments are fused by tagmosis into tagmata § Exoskeleton made of chitin § Secreted by epidermis § Molting (Ecdysis à Ecdysozoa) § Exoskeleton shed regularly during life or only during larval development § No cilia in larvae or adults v Most successful in terms of diversity and sheer numbers § >1,000,000 species! Arthropod Origins v The arthropod body plan consists of a segmented body, hard exoskeleton, and jointed appendages v This body plan dates to the Cambrian explosion (535–525 million years ago) v Early arthropods show little variation from segment to segment Phylum Arthropoda – general body plan v Body divided into segments (somites) § Regionally fused into specialized groups by tagmosis (i.e., 5 segments form head) § Internal cavity à hemocoel (not coelom!) § No internal segmentation (no septa – contrast w/annelids) v Each body segment has a pair of jointed appendages Tagmosis allows for specialization & diversity v Diversity of body form possible because of specialization of segments, regions and appendages v Tagmosis = segments grouped together § Specialized for particular functions for greater efficiency v Head, thorax and abdomen are tagmata § Regions specialized for performing different tasks Exoskeleton Structure v Cuticle forms well-developed exoskeleton made up of plates (sclerites) § Growth by ecdysis (hormone-induced molting) v Epidermis is a single layer of epithelial cells that secrete the cuticle in layers § Outer layer = epicuticle, with water-repellant hydrophobic layers § Inner layer = procuticle, protein & chitin § Procuticle is hardened by 1. -
Ultrastructure, Histochemistry, and Mineralization Patterns in the Ecdysial Suture of the Blue Crab, Callinectes Sapidus
Microsc. Microanal. 11, 479–499, 2005 / DOI: 10.1017 S1431927605050555 Microscopy AND Microanalysis © MICROSCOPY SOCIETY OF AMERICA 2005 Ultrastructure, Histochemistry, and Mineralization Patterns in the Ecdysial Suture of the Blue Crab, Callinectes sapidus Carolina Priester, Richard M. Dillaman,* and D. Mark Gay Department of Biological Sciences, University of North Carolina at Wilmington, Wilmington, NC 28403-5915, USA Abstract: The ecdysial suture is the region of the arthropod exoskeleton that splits to allow the animal to emerge during ecdysis. We examined the morphology and composition of the intermolt and premolt suture of the blue crab using light microscopy and scanning electron microscopy. The suture could not be identified by routine histological techniques; however 3 of 22 fluorescein isothiocyanate-labeled lectins tested ~Lens culinaris agglutinin, Vicia faba agglutinin, and Pisum sativum agglutinin! differentiated the suture, binding more intensely to the suture exocuticle and less intensely to the suture endocuticle. Back-scattered electron ~BSE! and secondary electron observations of fracture surfaces of intermolt cuticle showed less mineralized regions in the wedge-shaped suture as did BSE analysis of premolt and intermolt resin-embedded cuticle. The prism regions of the suture exocuticle were not calcified. X-ray microanalysis of both the endocuticle and exocuticle demonstrated that the suture was less calcified than the surrounding cuticle with significantly lower magnesium and phosphorus concentrations, potentially making its mineral more soluble. The presence or absence of a glycoprotein in the organic matrix, the extent and composition of the mineral deposited, and the thickness of the cuticle all likely contribute to the suture being removed by molting fluid, thereby ensuring successful ecdysis. -
Nguyen Vienny Ug Thesis Signed.Pdf (2.436Mb)
ABSTRACT Insect exoskeleton is an interesting and complex system that plays both structural and functional roles. It is a composite that consists of many different types of materials and shapes that vary based on its local function. This review of exoskeleton micro- and macro-structure addresses the performance of exoskeleton based on these two factors. Exoskeleton is a composite whose material properties are dependent on its constituents and fiber orientation. At the macro-structural level, exoskeleton’s shape can play many roles in local structural and motional functions. The lessons from this review are examined and considered for potential applications in the fields of material fabrication and development and in microrobotics. i ACKNOWLEDGEMENTS I give thanks to my family and friends for getting me to where I am today – for believing when they shouldn’t have. I am grateful for Dr. Lilly, my advisor, for his support and guidance. I am thankful for David, my fiancé, for his love. ii TABLE OF CONTENTS ABSTRACT ......................................................................................................................... i ACKNOWLEDGEMENTS ................................................................................................ ii TABLE OF CONTENTS ................................................................................................... iii LIST OF FIGURES ............................................................................................................ v LIST OF TABLES ........................................................................................................... -
Kein Folientitel
Structure and texture analysis of chitin-bio- Microstructure Physics and nanocomposites using synchrotron radiation Metal Forming Prof. Dr. D. Raabe Max-Planck-Institut für A. Al-Sawalmih, P. Romano, C. Sachs, D. Raabe Eisenforschung GmbH Düsseldorf/Germany The exoskeleton (shell) of arthropods typically consists of a biological chitin-based nano-composite associated with inorganic fraction. Chitin which is a linear polysaccharide is the second most abundant polysaccharide in the world after cellulose. It has basically the same structure as cellulose; the chains in both polymers are (beta)1-4 linked, but the difference is that chitin has an acetyl-amide group NH-CH3CO instead of the hydroxyl group (alcohol) in cellulose. Chitin occurs in the form of fibres in the shells of arthropods like crustacea and insects (α-chitin), and also in the loligo pen of the squids (β-chitin). In arthropod exoskeletons α-chitin fibrils of about 3 nm diameter are surrounded by a protein helix of about 2 nm thickness. These chitin-protein composite ligaments which form a crystalline lattice structure act as the main structural element of the arthropod exoskeleton (see fig.1). Depending on the type of arthropods the exoskeleton contains different fractions of biomineral precipitates such as calcite (CaCO3) and magnesian calcite (Mg0.1Ca0.9CO3). The crystalline particles control the hardness of the material. Chitin-based nano-composites with precipitate biominerals provide both considerable mechanical strength at a very small weight and functional flexibility such as required for living species. Chitin is available in huge amounts as a natural resource from arthropods such as shrimps and related crustacea.