Golgi Self-Correction Generates Bioequivalent Glycans to Preserve
Total Page:16
File Type:pdf, Size:1020Kb
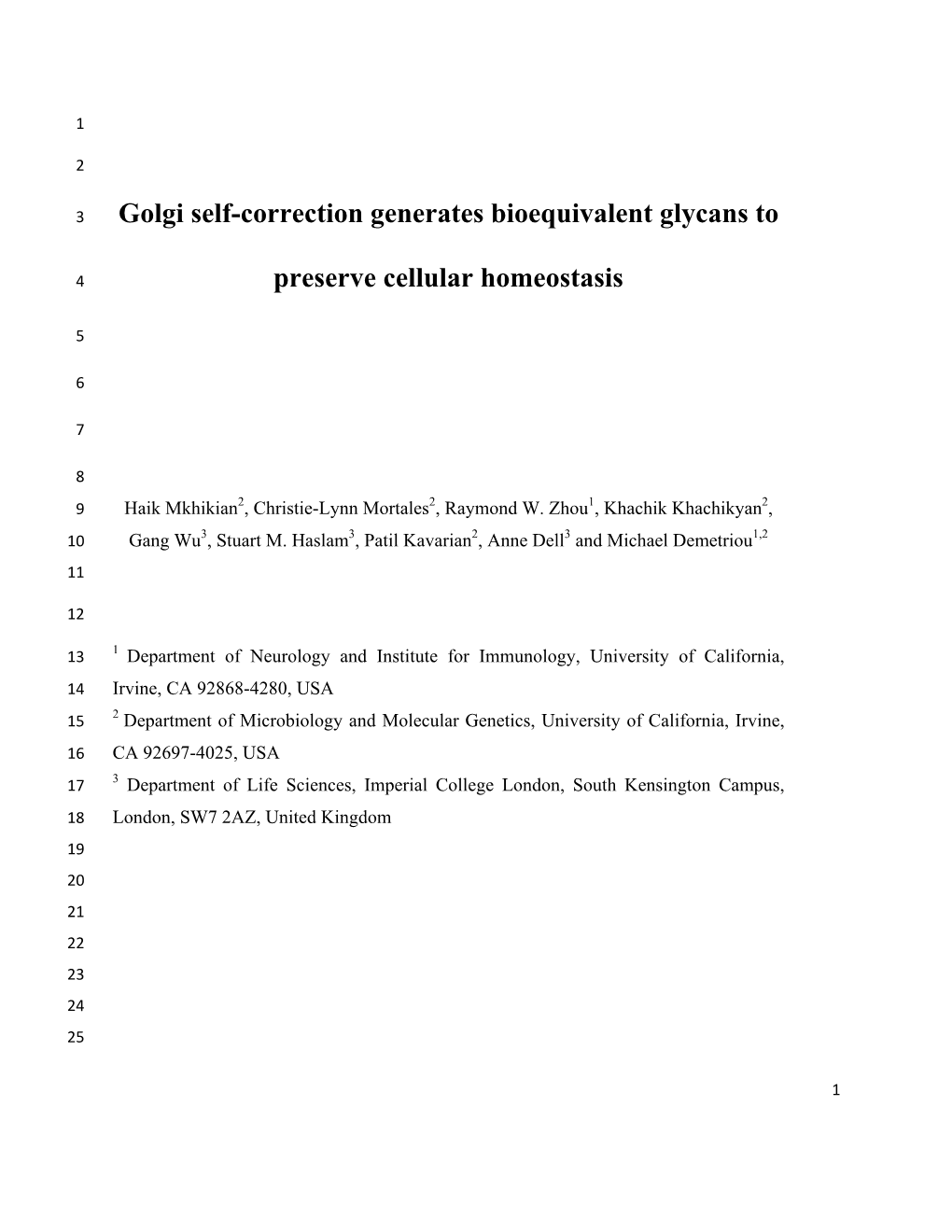
Load more
Recommended publications
-
Characterization of Genomic Copy Number Variation in Mus Musculus Associated with the Germline of Inbred and Wild Mouse Populations, Normal Development, and Cancer
Western University Scholarship@Western Electronic Thesis and Dissertation Repository 4-18-2019 2:00 PM Characterization of genomic copy number variation in Mus musculus associated with the germline of inbred and wild mouse populations, normal development, and cancer Maja Milojevic The University of Western Ontario Supervisor Hill, Kathleen A. The University of Western Ontario Graduate Program in Biology A thesis submitted in partial fulfillment of the equirr ements for the degree in Doctor of Philosophy © Maja Milojevic 2019 Follow this and additional works at: https://ir.lib.uwo.ca/etd Part of the Genetics and Genomics Commons Recommended Citation Milojevic, Maja, "Characterization of genomic copy number variation in Mus musculus associated with the germline of inbred and wild mouse populations, normal development, and cancer" (2019). Electronic Thesis and Dissertation Repository. 6146. https://ir.lib.uwo.ca/etd/6146 This Dissertation/Thesis is brought to you for free and open access by Scholarship@Western. It has been accepted for inclusion in Electronic Thesis and Dissertation Repository by an authorized administrator of Scholarship@Western. For more information, please contact [email protected]. Abstract Mus musculus is a human commensal species and an important model of human development and disease with a need for approaches to determine the contribution of copy number variants (CNVs) to genetic variation in laboratory and wild mice, and arising with normal mouse development and disease. Here, the Mouse Diversity Genotyping array (MDGA)-approach to CNV detection is developed to characterize CNV differences between laboratory and wild mice, between multiple normal tissues of the same mouse, and between primary mammary gland tumours and metastatic lung tissue. -
B4GALT2 Rabbit Pab
Leader in Biomolecular Solutions for Life Science B4GALT2 Rabbit pAb Catalog No.: A17573 Basic Information Background Catalog No. This gene is one of seven beta-1,4-galactosyltransferase (beta4GalT) genes. They A17573 encode type II membrane-bound glycoproteins that appear to have exclusive specificity for the donor substrate UDP-galactose; all transfer galactose in a beta1,4 linkage to Observed MW similar acceptor sugars: GlcNAc, Glc, and Xyl. Each beta4GalT has a distinct function in 42kDa the biosynthesis of different glycoconjugates and saccharide structures. As type II membrane proteins, they have an N-terminal hydrophobic signal sequence that directs Calculated MW the protein to the Golgi apparatus and which then remains uncleaved to function as a transmembrane anchor. By sequence similarity, the beta4GalTs form four groups: Category beta4GalT1 and beta4GalT2, beta4GalT3 and beta4GalT4, beta4GalT5 and beta4GalT6, and beta4GalT7. The enzyme encoded by this gene synthesizes N-acetyllactosamine in Primary antibody glycolipids and glycoproteins. Its substrate specificity is affected by alpha-lactalbumin but it is not expressed in lactating mammary tissue. Three transcript variants encoding Applications two different isoforms have been found for this gene. [provided by RefSeq, Jul 2011] WB,IHC Cross-Reactivity Human, Mouse, Rat Recommended Dilutions Immunogen Information WB 1:500 - 1:2000 Gene ID Swiss Prot 8704 O60909 IHC 1:100 - 1:200 Immunogen Recombinant fusion protein containing a sequence corresponding to amino acids 155-271 of human B4GALT2 (NP_085076.2). Synonyms B4Gal-T2;B4Gal-T3;beta4Gal-T2;B4GALT2 Contact Product Information 400-999-6126 Source Isotype Purification Rabbit IgG Affinity purification [email protected] www.abclonal.com.cn Storage Store at -20℃. -
B4GALT3 (B4GALT2) (NM 030587) Human Tagged ORF Clone Product Data
OriGene Technologies, Inc. 9620 Medical Center Drive, Ste 200 Rockville, MD 20850, US Phone: +1-888-267-4436 [email protected] EU: [email protected] CN: [email protected] Product datasheet for RC233988 B4GALT3 (B4GALT2) (NM_030587) Human Tagged ORF Clone Product data: Product Type: Expression Plasmids Product Name: B4GALT3 (B4GALT2) (NM_030587) Human Tagged ORF Clone Tag: Myc-DDK Symbol: B4GALT2 Synonyms: B4Gal-T2; B4Gal-T3; beta4Gal-T2 Vector: pCMV6-Entry (PS100001) E. coli Selection: Kanamycin (25 ug/mL) Cell Selection: Neomycin ORF Nucleotide >RC233988 representing NM_030587 Sequence: Red=Cloning site Blue=ORF Green=Tags(s) TTTTGTAATACGACTCACTATAGGGCGGCCGGGAATTCGTCGACTGGATCCGGTACCGAGGAGATCTGCC GCCGCGATCGCC ATGGCTGTGGAAGTCCAGGAGCAGTGGCCTTGTTTGCCAGCAGCCGGATGCCCGGGCCCACTGGGCGGGC CAGTGGCCGCCTGCGGGATGAGCAGACTGCTGGGGGGGACGCTGGAGCGCGTCTGCAAGGCTGTGCTCCT TCTCTGCCTGCTGCACTTCCTCGTGGCCGTCATCCTCTACTTTGACGTCTACGCCCAGCACCTGGCCTTC TTCAGCCGCTTCAGTGCCCGAGGCCCTGCCCATGCCCTCCACCCAGCTGCTAGCAGCAGCAGCAGCAGCA GCAACTGCTCCCGGCCCAACGCCACCGCCTCTAGCTCCGGGCTCCCTGAGGTCCCCAGTGCCCTGCCCGG TCCCACGGCTCCCACGCTGCCACCCTGTCCTGACTCGCCACCTGGTCTTGTGGGCAGACTGCTGATCGAG TTCACCTCACCCATGCCCCTGGAGCGGGTGCAGAGGGAGAACCCAGGCGTGCTCATGGGCGGCCGATACA CACCGCCCGACTGCACCCCAGCCCAGACGGTGGCGGTCATCATCCCCTTTAGACACCGGGAACACCACCT GCGCTACTGGCTCCACTATCTACACCCCATCTTGAGGCGGCAGCGGCTGCGCTACGGCGTCTATGTCATC AACCAGCATGGTGAGGACACCTTCAACCGGGCCAAGCTGCTTAACGTGGGCTTCCTAGAGGCGCTGAAGG AGGATGCCGCCTATGACTGCTTCATCTTCAGCGATGTGGACCTGGTCCCCATGGATGACCGCAACCTATA CCGCTGCGGCGACCAACCCCGCCACTTTGCCATTGCCATGGACAAGTTTGGCTTCCGGCTTCCCTATGCT -
Multiplexed Engineering Glycosyltransferase Genes in CHO Cells Via Targeted Integration for Producing Antibodies with Diverse Complex‑Type N‑Glycans Ngan T
www.nature.com/scientificreports OPEN Multiplexed engineering glycosyltransferase genes in CHO cells via targeted integration for producing antibodies with diverse complex‑type N‑glycans Ngan T. B. Nguyen, Jianer Lin, Shi Jie Tay, Mariati, Jessna Yeo, Terry Nguyen‑Khuong & Yuansheng Yang* Therapeutic antibodies are decorated with complex‑type N‑glycans that signifcantly afect their biodistribution and bioactivity. The N‑glycan structures on antibodies are incompletely processed in wild‑type CHO cells due to their limited glycosylation capacity. To improve N‑glycan processing, glycosyltransferase genes have been traditionally overexpressed in CHO cells to engineer the cellular N‑glycosylation pathway by using random integration, which is often associated with large clonal variations in gene expression levels. In order to minimize the clonal variations, we used recombinase‑mediated‑cassette‑exchange (RMCE) technology to overexpress a panel of 42 human glycosyltransferase genes to screen their impact on antibody N‑linked glycosylation. The bottlenecks in the N‑glycosylation pathway were identifed and then released by overexpressing single or multiple critical genes. Overexpressing B4GalT1 gene alone in the CHO cells produced antibodies with more than 80% galactosylated bi‑antennary N‑glycans. Combinatorial overexpression of B4GalT1 and ST6Gal1 produced antibodies containing more than 70% sialylated bi‑antennary N‑glycans. In addition, antibodies with various tri‑antennary N‑glycans were obtained for the frst time by overexpressing MGAT5 alone or in combination with B4GalT1 and ST6Gal1. The various N‑glycan structures and the method for producing them in this work provide opportunities to study the glycan structure‑and‑function and develop novel recombinant antibodies for addressing diferent therapeutic applications. -
Supplementary Data
Supplementary Fig. 1 A B Responder_Xenograft_ Responder_Xenograft_ NON- NON- Lu7336, Vehicle vs Lu7466, Vehicle vs Responder_Xenograft_ Responder_Xenograft_ Sagopilone, Welch- Sagopilone, Welch- Lu7187, Vehicle vs Lu7406, Vehicle vs Test: 638 Test: 600 Sagopilone, Welch- Sagopilone, Welch- Test: 468 Test: 482 Responder_Xenograft_ NON- Lu7860, Vehicle vs Responder_Xenograft_ Sagopilone, Welch - Lu7558, Vehicle vs Test: 605 Sagopilone, Welch- Test: 333 Supplementary Fig. 2 Supplementary Fig. 3 Supplementary Figure S1. Venn diagrams comparing probe sets regulated by Sagopilone treatment (10mg/kg for 24h) between individual models (Welsh Test ellipse p-value<0.001 or 5-fold change). A Sagopilone responder models, B Sagopilone non-responder models. Supplementary Figure S2. Pathway analysis of genes regulated by Sagopilone treatment in responder xenograft models 24h after Sagopilone treatment by GeneGo Metacore; the most significant pathway map representing cell cycle/spindle assembly and chromosome separation is shown, genes upregulated by Sagopilone treatment are marked with red thermometers. Supplementary Figure S3. GeneGo Metacore pathway analysis of genes differentially expressed between Sagopilone Responder and Non-Responder models displaying –log(p-Values) of most significant pathway maps. Supplementary Tables Supplementary Table 1. Response and activity in 22 non-small-cell lung cancer (NSCLC) xenograft models after treatment with Sagopilone and other cytotoxic agents commonly used in the management of NSCLC Tumor Model Response type -
Supplementary Table 2 Differentially Expressed Genes in PC3 Prostate Adenocarcinoma Cells 48 H After Transfection with Sirxfp1 Versus Sinc Control Sirna
Supplementary Table 2 Differentially expressed genes in PC3 prostate adenocarcinoma cells 48 h after transfection with siRXFP1 versus siNC control siRNA. Genes with P<0.05 are shown Average fold change PROBE_ID TargetID Title (siRXFP1/siNC) ILMN_1757406 HIST1H1C histone cluster 1, H1c 2.358875 ILMN_1691846 G0S2 G0/G1switch 2 2.148632 ILMN_1706505 COL5A1 collagen, type V, alpha 1 2.030442 ILMN_1715684 LAMB3 laminin, beta 3 1.891462 ILMN_1809402 MAN2A1 mannosidase, alpha, class 2A, member 1 1.843399 ILMN_1666503 DENND2A DENN/MADD domain containing 2A 1.794946 ILMN_1746465 FJX1 four jointed box 1 (Drosophila) 1.777726 ILMN_1688670 CDCP1 CUB domain containing protein 1 1.743697 ILMN_1708341 PDZK1 PDZ domain containing 1 1.740739 ILMN_1658702 HIST1H2BJ histone cluster 1, H2bj 1.621722 ILMN_1756777 HBEGF heparin-binding EGF-like growth factor 1.594607 ILMN_1727315 DENND1A DENN/MADD domain containing 1A 1.581216 CKLF-like MARVEL transmembrane domain ILMN_1705442 CMTM3 containing 3 1.529026 estrogen receptor binding site associated, ILMN_1729144 EBAG9 antigen, 9 1.507585 ILMN_1666507 PLAUR plasminogen activator, urokinase receptor 1.480579 plakophilin 1 (ectodermal dysplasia/skin ILMN_1663454 PKP1 fragility syndrome) 1.475808 ILMN_1706643 COL6A3 collagen, type VI, alpha 3 1.47301 ILMN_1795055 LRRC3 leucine rich repeat containing 3 1.471709 ILMN_1722845 RAB3B RAB3B, member RAS oncogene family 1.432296 ILMN_1691508 PLAUR plasminogen activator, urokinase receptor 1.423904 ILMN_1703531 EDG3 sphingosine-1-phosphate receptor 3 1.421666 ILMN_1803728 -
Supplementary Data
Progressive Disease Signature Upregulated probes with progressive disease U133Plus2 ID Gene Symbol Gene Name 239673_at NR3C2 nuclear receptor subfamily 3, group C, member 2 228994_at CCDC24 coiled-coil domain containing 24 1562245_a_at ZNF578 zinc finger protein 578 234224_at PTPRG protein tyrosine phosphatase, receptor type, G 219173_at NA NA 218613_at PSD3 pleckstrin and Sec7 domain containing 3 236167_at TNS3 tensin 3 1562244_at ZNF578 zinc finger protein 578 221909_at RNFT2 ring finger protein, transmembrane 2 1552732_at ABRA actin-binding Rho activating protein 59375_at MYO15B myosin XVB pseudogene 203633_at CPT1A carnitine palmitoyltransferase 1A (liver) 1563120_at NA NA 1560098_at AKR1C2 aldo-keto reductase family 1, member C2 (dihydrodiol dehydrogenase 2; bile acid binding pro 238576_at NA NA 202283_at SERPINF1 serpin peptidase inhibitor, clade F (alpha-2 antiplasmin, pigment epithelium derived factor), m 214248_s_at TRIM2 tripartite motif-containing 2 204766_s_at NUDT1 nudix (nucleoside diphosphate linked moiety X)-type motif 1 242308_at MCOLN3 mucolipin 3 1569154_a_at NA NA 228171_s_at PLEKHG4 pleckstrin homology domain containing, family G (with RhoGef domain) member 4 1552587_at CNBD1 cyclic nucleotide binding domain containing 1 220705_s_at ADAMTS7 ADAM metallopeptidase with thrombospondin type 1 motif, 7 232332_at RP13-347D8.3 KIAA1210 protein 1553618_at TRIM43 tripartite motif-containing 43 209369_at ANXA3 annexin A3 243143_at FAM24A family with sequence similarity 24, member A 234742_at SIRPG signal-regulatory protein gamma -
The Molecular Signature of the Stroma Response in Prostate
RESEARCH ARTICLE The Molecular Signature of the Stroma Response in Prostate Cancer-Induced Osteoblastic Bone Metastasis Highlights Expansion of Hematopoietic and Prostate Epithelial Stem Cell Niches Berna C. O¨ zdemir1., Janine Hensel1., Chiara Secondini1, Antoinette Wetterwald1, Ruth Schwaninger1, Achim Fleischmann2, Wolfgang Raffelsberger3, OPEN ACCESS Olivier Poch4, Mauro Delorenzi5, Ramzi Temanni6, Ian G. Mills7, Gabri van der Citation: O¨ zdemir BC, Hensel J, Secondini C, Pluijm8, George N. Thalmann1, Marco G. Cecchini1* Wetterwald A, Schwaninger R, et al. (2014) The Molecular Signature of the Stroma Response in 1. Urology Research Laboratory, Department of Urology and Department of Clinical Research, University of Prostate Cancer-Induced Osteoblastic Bone Bern, Bern, Switzerland, 2. Institute of Pathology, University of Bern, Bern, Switzerland, 3. Institut Ge´ne´tique Metastasis Highlights Expansion of Hematopoietic Biologie Mole´culaire Cellulaire (IGBMC), Strasbourg, France, 4. ICube UMR7357, University of Strasbourg, and Prostate Epithelial Stem Cell Niches. PLoS Strasbourg, France, 5. Ludwig Center for Cancer Research, Department of Oncology, University of Lausanne ONE 9(12): e114530. doi:10.1371/journal.pone. and Swiss Institute of Bioinformatics (SIB), Lausanne, Switzerland, 6. Biomedical Informatics Division, Sidra 0114530 Medical and Research Center, Doha, Qatar, 7. Prostate Cancer Research Group, Norway Centre for Editor: Adriano Angelucci, University of L9Aquila, Molecular Medicine (NCMM), University of Oslo, Oslo, Norway, -
Clinical Impact of Copy Number Variation Changes in Bladder Cancer Samples
EXPERIMENTAL AND THERAPEUTIC MEDICINE 22: 901, 2021 Clinical impact of copy number variation changes in bladder cancer samples VICTORIA SPASOVA1, BORIS MLADENOV2, SIMEON RANGELOV3, ZORA HAMMOUDEH1, DESISLAVA NESHEVA1, DIMITAR SERBEZOV1, RADA STANEVA1,4, SAVINA HADJIDEKOVA1,4, MIHAIL GANEV1, LUBOMIR BALABANSKI1,5, RADOSLAVA VAZHAROVA5,6, CHAVDAR SLAVOV3, DRAGA TONCHEVA1 and OLGA ANTONOVA1 1Department of Medical Genetics, Medical University‑Sofia, 1431 Sofia;2 Department of Urology, UMBALSM N.I. Pirogov, 1606 Sofia; 3Department of Urology, Tsaritsa Yoanna University Hospital, 1527 Sofia; 4Medical Genetics Laboratory, Nadezhda Women's Health Hospital, 1373 Sofia; 5Medical Genetics Laboratory, GARH Malinov, 1680 Sofia; 6Department of Biology, Medical Genetics and Microbiology, Faculty of Medicine, Sofia University St. Kliment Ohridski, 1407 Sofia, Bulgaria Received November 30, 2019; Accepted February 18, 2021 DOI: 10.3892/etm.2021.10333 Abstract. The aim of the present study was to detect copy uroepithelial tumours may lay a foundation for implementing number variations (CNVs) related to tumour progression and molecular CNV profiling of bladder tumours as part of a metastasis of urothelial carcinoma through whole‑genome routine progression risk estimation strategy, thus expanding scanning. A total of 30 bladder cancer samples staged from the personalized therapeutic approach. pTa to pT4 were included in the study. DNA was extracted from freshly frozen tissue via standard phenol‑chloroform extraction Introduction and CNV analysis was performed on two alternative platforms (CytoChip Oligo aCGH, 4x44K and Infinium OncoArray‑500K The most successful approach to treating a disease has BeadChip; Illumina, Inc.). Data were analysed with BlueFuse always been etiological therapy. In the case of bladder Multi software and Karyostudio, respectively. -
Single Cell Derived Clonal Analysis of Human Glioblastoma Links
SUPPLEMENTARY INFORMATION: Single cell derived clonal analysis of human glioblastoma links functional and genomic heterogeneity ! Mona Meyer*, Jüri Reimand*, Xiaoyang Lan, Renee Head, Xueming Zhu, Michelle Kushida, Jane Bayani, Jessica C. Pressey, Anath Lionel, Ian D. Clarke, Michael Cusimano, Jeremy Squire, Stephen Scherer, Mark Bernstein, Melanie A. Woodin, Gary D. Bader**, and Peter B. Dirks**! ! * These authors contributed equally to this work.! ** Correspondence: [email protected] or [email protected]! ! Supplementary information - Meyer, Reimand et al. Supplementary methods" 4" Patient samples and fluorescence activated cell sorting (FACS)! 4! Differentiation! 4! Immunocytochemistry and EdU Imaging! 4! Proliferation! 5! Western blotting ! 5! Temozolomide treatment! 5! NCI drug library screen! 6! Orthotopic injections! 6! Immunohistochemistry on tumor sections! 6! Promoter methylation of MGMT! 6! Fluorescence in situ Hybridization (FISH)! 7! SNP6 microarray analysis and genome segmentation! 7! Calling copy number alterations! 8! Mapping altered genome segments to genes! 8! Recurrently altered genes with clonal variability! 9! Global analyses of copy number alterations! 9! Phylogenetic analysis of copy number alterations! 10! Microarray analysis! 10! Gene expression differences of TMZ resistant and sensitive clones of GBM-482! 10! Reverse transcription-PCR analyses! 11! Tumor subtype analysis of TMZ-sensitive and resistant clones! 11! Pathway analysis of gene expression in the TMZ-sensitive clone of GBM-482! 11! Supplementary figures and tables" 13" "2 Supplementary information - Meyer, Reimand et al. Table S1: Individual clones from all patient tumors are tumorigenic. ! 14! Fig. S1: clonal tumorigenicity.! 15! Fig. S2: clonal heterogeneity of EGFR and PTEN expression.! 20! Fig. S3: clonal heterogeneity of proliferation.! 21! Fig. -
B-1,4-Galactosyltransferase III Enhances Invasive Phenotypes Via B1-Integrin and Predicts Poor Prognosis in Neuroblastoma
Published OnlineFirst February 26, 2013; DOI: 10.1158/1078-0432.CCR-12-2367 Clinical Cancer Human Cancer Biology Research b-1,4-Galactosyltransferase III Enhances Invasive Phenotypes Via b1-Integrin and Predicts Poor Prognosis in Neuroblastoma Hsiu-Hao Chang1, Chia-Hua Chen5, Chih-Hsing Chou5, Yung-Feng Liao6, Miao-Juei Huang5,9, Ya-Hsin Chen5,9, Wei-Jen Wang5,9, John Huang4, Ji-Shiang Hung4, Wan-Ling Ho7,10, Yung-Ming Jeng2, Mei-Ieng Che5, Hsinyu Lee4,8, Meng-Yao Lu1, Yung-Li Yang1,3, Shiann-Tarng Jou1, Dong-Tsamn Lin1,3, Kai-Hsin Lin1, Wen-Ming Hsu4,9, and Min-Chuan Huang5,9 Abstract Purpose: Neuroblastoma (NB) is a neural crest-derived tumor that commonly occurs in childhood. b-1,4-Galactosyltransferase III (B4GALT3) is highly expressed in human fetal brain and is responsible for the generation of poly-N-acetyllactosamine, which plays a critical role in tumor progression. We therefore investigated the expression and role of B4GALT3 in NB. Experimental Design: We examined B4GALT3 expression in tumor specimens from 101 NB patients by immunohistochemistry and analyzed the correlation between B4GALT3 expression and clinicopathologic factors or survival. The functional role of B4GALT3 expression was investigated by overexpression or knockdown of B4GALT3 in NB cells for in vitro and in vivo studies. Results: We found that B4GALT3 expression correlated with advanced clinical stages (P ¼ 0.040), unfavorable Shimada histology (P < 0.001), and lower survival rate (P < 0.001). Multivariate analysis showed that B4GALT3 expression is an independent prognostic factor for poor survival of NB patients. B4GALT3 overexpression increased migration, invasion, and tumor growth of NB cells, whereas B4GALT3 knockdown suppressed the malignant phenotypes of NB cells. -
Integrated Bioinformatic Analysis Identifies UBE2Q1 As a Potential
Topno et al. BMC Cancer (2021) 21:220 https://doi.org/10.1186/s12885-021-07928-z RESEARCH ARTICLE Open Access Integrated bioinformatic analysis identifies UBE2Q1 as a potential prognostic marker for high grade serous ovarian cancer Rachel Topno1,2†, Ibha Singh3†, Manoj Kumar1* and Pallavi Agarwal3* Abstract Background: High grade serous ovarian cancer (HGSOC) accounts for nearly 60% of total cases of epithelial ovarian cancer. It is the most aggressive subtype, which shows poor prognosis and low patient survival. For better management of HGSOC patients, new prognostic biomarkers are required to facilitate improved treatment strategies and ensure suitable healthcare decisions. Methods: We performed genome wide expression analysis of HGSOC patient samples to identify differentially expressed genes (DEGs) using R based Limma package, Clust and other statistical tools. The identified DEGs were subjected to weighted gene co-expression network analysis (WGCNA) to identify co-expression patterns of relevant genes. Module trait and gene ontology analyses were performed to establish important gene co-expression networks and their biological functions. Overlapping the most relevant DEG cluster 4 with prominent WGCNA cyan module identified strongest correlation of UBE2Q1 with ovarian cancer and its prognostic significance on survival probability of ovarian cancer patients was investigated. The predictive value of UBE2Q1 as a potential biomarker was analysed by correlating its expression with 12-months relapse free survival of patients in response to platin/ taxane, the standard first-line chemotherapy for ovarian cancer, and analysing area under the ROC curve. Results: An integrated gene expression analysis and WGCNA, identified UBE2Q1 as a potential prognostic marker associated with poor relapse-free survival and response outcome to platin/taxane treatment of patients with high grade serous ovarian cancer.