FINAL REPORT Pines Vs
Total Page:16
File Type:pdf, Size:1020Kb
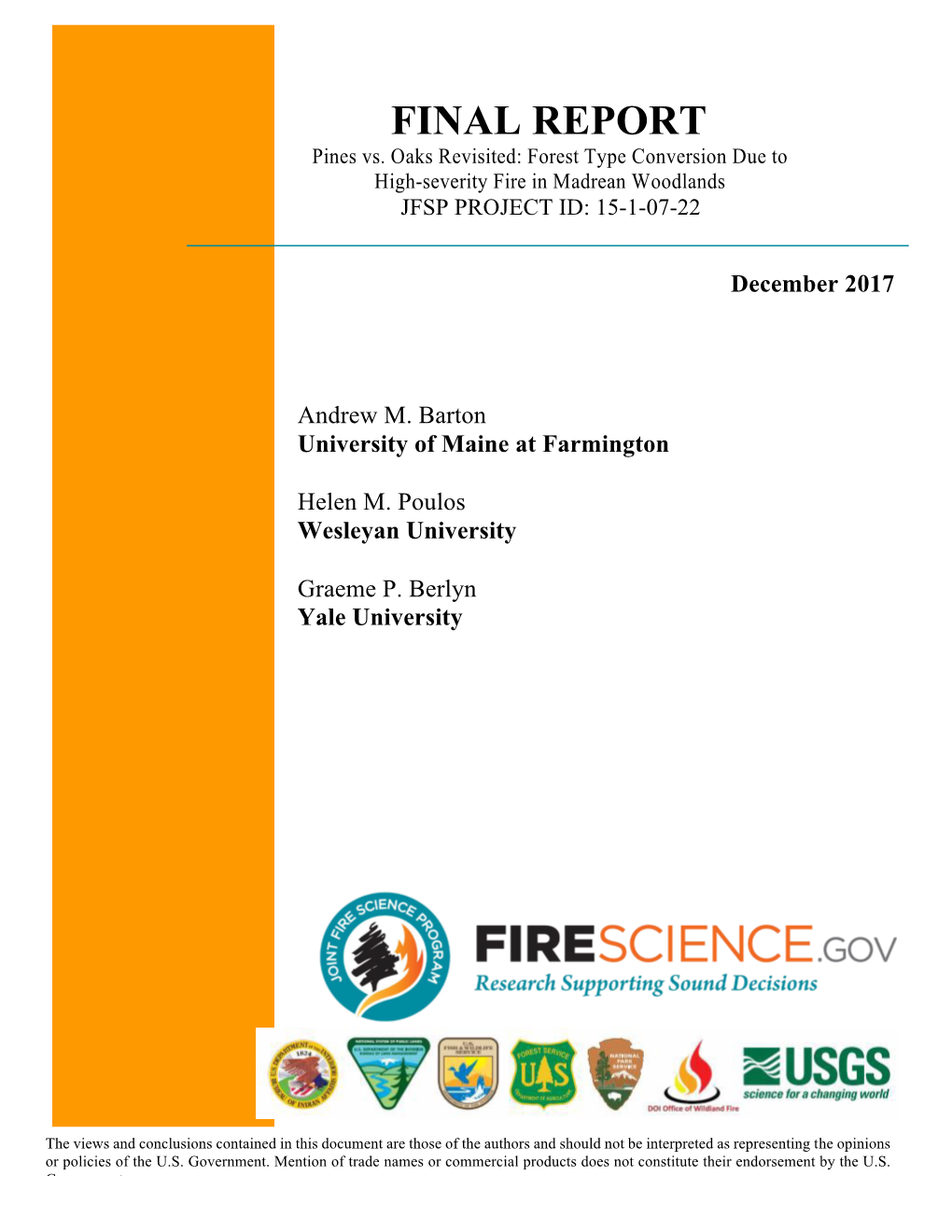
Load more
Recommended publications
-
Texas Big Tree Registry a List of the Largest Trees in Texas Sponsored by Texas a & M Forest Service
Texas Big Tree Registry A list of the largest trees in Texas Sponsored by Texas A & M Forest Service Native and Naturalized Species of Texas: 320 ( D indicates species naturalized to Texas) Common Name (also known as) Latin Name Remarks Cir. Threshold acacia, Berlandier (guajillo) Senegalia berlandieri Considered a shrub by B. Simpson 18'' or 1.5 ' acacia, blackbrush Vachellia rigidula Considered a shrub by Simpson 12'' or 1.0 ' acacia, Gregg (catclaw acacia, Gregg catclaw) Senegalia greggii var. greggii Was named A. greggii 55'' or 4.6 ' acacia, Roemer (roundflower catclaw) Senegalia roemeriana 18'' or 1.5 ' acacia, sweet (huisache) Vachellia farnesiana 100'' or 8.3 ' acacia, twisted (huisachillo) Vachellia bravoensis Was named 'A. tortuosa' 9'' or 0.8 ' acacia, Wright (Wright catclaw) Senegalia greggii var. wrightii Was named 'A. wrightii' 70'' or 5.8 ' D ailanthus (tree-of-heaven) Ailanthus altissima 120'' or 10.0 ' alder, hazel Alnus serrulata 18'' or 1.5 ' allthorn (crown-of-thorns) Koeberlinia spinosa Considered a shrub by Simpson 18'' or 1.5 ' anacahuita (anacahuite, Mexican olive) Cordia boissieri 60'' or 5.0 ' anacua (anaqua, knockaway) Ehretia anacua 120'' or 10.0 ' ash, Carolina Fraxinus caroliniana 90'' or 7.5 ' ash, Chihuahuan Fraxinus papillosa 12'' or 1.0 ' ash, fragrant Fraxinus cuspidata 18'' or 1.5 ' ash, green Fraxinus pennsylvanica 120'' or 10.0 ' ash, Gregg (littleleaf ash) Fraxinus greggii 12'' or 1.0 ' ash, Mexican (Berlandier ash) Fraxinus berlandieriana Was named 'F. berlandierana' 120'' or 10.0 ' ash, Texas Fraxinus texensis 60'' or 5.0 ' ash, velvet (Arizona ash) Fraxinus velutina 120'' or 10.0 ' ash, white Fraxinus americana 100'' or 8.3 ' aspen, quaking Populus tremuloides 25'' or 2.1 ' baccharis, eastern (groundseltree) Baccharis halimifolia Considered a shrub by Simpson 12'' or 1.0 ' baldcypress (bald cypress) Taxodium distichum Was named 'T. -
Oaks of the Wild West Inventory Page 1 Nursery Stock Feb, 2016
Oaks of the Wild West Inventory Nursery Stock Legend: AZ = Arizona Nursery TX = Texas Nursery Feb, 2016 *Some species are also available in tube sizes Pine Trees Scientific Name 1G 3/5G 10G 15 G Aleppo Pine Pinus halapensis AZ Afghan Pine Pinus elderica AZ Apache Pine Pinus engelmannii AZ Chinese Pine Pinus tabulaeformis AZ Chihuahua Pine Pinus leiophylla Cluster Pine Pinus pinaster AZ Elderica Pine Pinus elderica AZ AZ Italian Stone Pine Pinus pinea AZ Japanese Black Pine Pinus thunbergii Long Leaf Pine Pinus palustris Mexican Pinyon Pine Pinus cembroides AZ Colorado Pinyon Pine Pinus Edulis AZ Ponderosa Pine Pinus ponderosa AZ Scotch Pine Pinus sylvestre AZ Single Leaf Pine Pinus monophylla AZ Texas Pine Pinus remota AZ, TX Common Trees Scientific Name 1G 3/5G 10G 15 G Arizona Sycamore Platanus wrightii ** Ash, Arizona Fraxinus velutina AZ AZ Black Walnut, Arizona Juglans major AZ AZ Black Walnut, Texas Juglans microcarpa TX Black Walnut juglans nigra AZ, TX Big Tooth Maple Acer grandidentatum AZ Carolina Buckthorn Rhamnus caroliniana TX Chitalpa Chitalpa tashkentensis AZ Crabapple, Blanco Malus ioensis var. texana Cypress, Bald Taxodium distichum AZ Desert Willow Chillopsis linearis AZ AZ Elm, Cedar Ulmus crassifolia TX TX Ginko Ginkgo biloba TX Hackberry, Canyon Celtis reticulata AZ AZ AZ Hackberry, Common Celtis occidentalis TX Maple (Sugar) Acer saccharum AZ AZ Mexican Maple Acer skutchii AZ Mexican Sycamore Platanus mexicana ** Mimosa, fragrant Mimosa borealis Page 1 Oaks of the Wild West Inventory Pistache (Red Push) Pistacia -
Universidad Autónoma Agraria Antonio Narro
UNIVERSIDAD AUTÓNOMA AGRARIA ANTONIO NARRO DIVISIÓN DE AGRONOMÍA Pinus arizonica Engelm. PRESENTA: SERGIO AMILCAR CANUL TUN MONOGRAFÍA Presentada como requisito parcial para Obtener el título de: INGENIERO FORESTAL Buenavista, Saltillo, Coahuila, México Mayo de 2005 UNIVERSIDAD AUTÓNOMA AGRARIA ANTONIO NARRO DIVISIÓN DE AGRONOMÍA DEPARTAMENTO FORESTAL Pinus arizonica Engelm. MONOGRAFÍA Que somete a consideración del H. Jurado calificador como requisito parcial para obtener el título de: INGENIERO FORESTAL PRESENTA SERGIO AMILCAR CANUL TUN APROBADA PRESIDENTE DEL JURADO COORDINADOR DE LA DIVISIÓN DE AGRONOMÍA _____________________________ ________________________________ M. C. CELESTINO FLORES LÓPEZ M. C. ARNOLDO OYERVIDES GARCÍA Buenavista, Saltillo, Coahuila, México Mayo de 2005 UNIVERSIDAD AUTÓNOMA AGRARIA ANTONIO NARRO DIVISIÓN DE AGRONOMÍA DEPARTAMENTO FORESTAL Pinus arizonica Engelm. MONOGRAFÍA Que somete a consideración del H. Jurado calificador como requisito parcial para obtener el título de: INGENIERO FORESTAL PRESENTA SERGIO AMILCAR CANUL TUN PRESIDENTE DEL JURADO _____________________________ M. C. CELESTINO FLORES LÓPEZ PRIMER SINODAL SEGUNDO SINODAL _______________________________ ___________________________________ Dr. MIGUEL ÁNGEL CAPÓ ARTEAGA Dr. JOSÉ ÁNGEL VILLARREAL QUINTANILLA Buenavista, Saltillo, Coahuila, México Mayo de 2005 DEDICATORIA A mis grandes amores: Leydi Abigail Cob Chan Nayvi Abisai Canul Cob Por haber llegado a mi vida y formar parte de mi, son un gran motivo para triunfar y salir adelante en medio de una gran tempestad. A mis padres: Ramón Cruz Canul Vázquez Lucila Tún Cahuich. Por su gran sacrificio realizado, por la confianza que me brindaron y por ser mis mejores amigos. Siempre tuve sus consejos, ayuda en el momento más crítico y comprensión. A mis hermanos: Claudio Ezequiel, por aceptar ser mi amigo, por su compañía y apoyo durante mi carrera y en este trabajo. -
Degree of Hybridization in Seed Stands of Pinus Engelmannii Carr
RESEARCH ARTICLE Degree of Hybridization in Seed Stands of Pinus engelmannii Carr. In the Sierra Madre Occidental, Durango, Mexico Israel Jaime Ávila-Flores1, José Ciro Hernández-Díaz2, Maria Socorro González-Elizondo3, José Ángel Prieto-Ruíz4, Christian Wehenkel2* 1 Doctorado Institucional en Ciencias Agropecuarias y Forestales, Universidad Juárez del Estado de Durango, Apdo, Postal 741, Zona Centro, Durango, Durango, México, C.P., 34000, 2 Instituto de Silvicultura e Industria de la Madera, Universidad Juárez del Estado de Durango, Apdo, Postal 741, Zona Centro, Durango, Durango, México, C.P., 34000, 3 CIIDIR Unidad Durango, Instituto Politécnico Nacional, Sigma 119 Fracc, 20 de Noviembre II, Durango, Durango, México, C.P., 34220, 4 Facultad de Ciencias Forestales, Universidad Juárez del Estado de Durango, Blv. Durango y Ave, Papaloapan s/n. Col. Valle del Sur, Durango, Durango, México, C.P., 34120 * [email protected] Abstract Hybridization is an important evolutionary force, because interspecific gene transfer can introduce more new genetic material than is directly generated by mutations. Pinus engel- OPEN ACCESS mannii Carr. is one of the nine most common pine species in the pine-oak forest ecoregion Citation: Ávila-Flores IJ, Hernández-Díaz JC, in the state of Durango, Mexico. This species is widely harvested for lumber and is also González-Elizondo MS, Prieto-Ruíz JÁ, Wehenkel C used in reforestation programmes. Interspecific hybrids between P.engelmannii and Pinus (2016) Degree of Hybridization in Seed Stands of arizonica Engelm. have been detected by morphological analysis. The presence of hybrids Pinus engelmannii Carr. In the Sierra Madre in P. engelmannii seed stands may affect seed quality and reforestation success. -
Previously Unrecorded Damage to Oak, Quercus Spp., in Southern California by the Goldspotted Oak Borer, Agrilus Coxalis Waterhouse (Coleoptera: Buprestidae) 1 2 TOM W
THE PAN-PACIFIC ENTOMOLOGIST 84(4):288–300, (2008) Previously unrecorded damage to oak, Quercus spp., in southern California by the goldspotted oak borer, Agrilus coxalis Waterhouse (Coleoptera: Buprestidae) 1 2 TOM W. COLEMAN AND STEVEN J. SEYBOLD 1USDA Forest Service-Forest Health Protection, 602 S. Tippecanoe Ave., San Bernardino, California 92408 Corresponding author: e-mail: [email protected] 2USDA Forest Service-Pacific Southwest Research Station, Chemical Ecology of Forest Insects, 720 Olive Dr., Suite D, Davis, California 95616 e-mail: [email protected] Abstract. A new and potentially devastating pest of oaks, Quercus spp., has been discovered in southern California. The goldspotted oak borer, Agrilus coxalis Waterhouse (Coleoptera: Buprestidae), colonizes the sapwood surface and phloem of the main stem and larger branches of at least three species of Quercus in San Diego Co., California. Larval feeding kills patches and strips of the phloem and cambium resulting in crown die back followed by mortality. In a survey of forest stand conditions at three sites in this area, 67% of the Quercus trees were found with external or internal evidence of A. coxalis attack. The literature and known distribution of A. coxalis are reviewed, and similarities in the behavior and impact of this species with other tree-killing Agrilus spp. are discussed. Key Words. Agrilus coxalis, California, flatheaded borer, introduced species, oak mortality, Quercus agrifolia, Quercus chrysolepis, Quercus kelloggii, range expansion. INTRODUCTION Extensive mortality of coast live oak, Quercus agrifolia Ne´e (Fagaceae), Engelmann oak, Quercus engelmannii Greene, and California black oak, Q. kelloggii Newb., has occurred since 2002 on the Cleveland National Forest (CNF) in San Diego Co., California. -
Arctostaphylos Pungens Woodland
54. [Quercus arizonica - Quercus emoryi] / Arctostaphylos pungens Woodland Association (P) [Arizona white oak - Emory oak] / Pointleaf manzanita Woodland Association (P) This woodland is characterized by a variably dense (20–40% cover) canopy stratum (2–5 m) dominated by Arizona white oak (Quercus Common species arizonica) and Emory oak (Quercus emoryi) with a similarly dense • Quercus arizonica (20–40% cover) subcanopy (0.5–2 m) dominated by manzanita (Arcto- • Quercus emoryi staphylos sp.), mainly pointleaf manzanita (Arctostaphylos pungens). The • Arctostaphylos pungens oak species provide similar cover (10%) as co-dominants or with either one becoming the sole dominant. Both species can range from small (<2 m), shrubby trees to single-stem individuals with heights up to 5 meters. Alligator juniper (Juniperus deppeana) and border pinyon (Pinus discolor) are sparse (1%) associates. Pointleaf manzanita (A. pungens) provides around 20+% cover throughout the community, with some areas reaching up to 40%. Other documented species include Pringle’s manzanita (Arctostaphylos pringlei), Wright’s silktassel (Garrya wrightii), yucca (Yucca madrensis), beargrass (Nolina microcarpa), California brickellbush (Brickellia californica), Fendler’s ceanothus (Ceanothus fendleri), and bullgrass (Muhlenbergia emersleyi). This community is contained within a two-association map class that covers 1.3% (362 ha/894 ac) of the Rincon Mountain District and occurs throughout the high slopes of Tanque Verde and Heartbreak ridges, often within the boundaries of historic fire events, specifically the 1989 Chiva Fire. It is primarily present on Rincon Mountain District, Saguaro National Park Rincon Mountain District, Saguaro moderately steep (20–40%) mountain backslopes from 1,450 to 1,650 meters (4,757–5,413 ft). -
Ecosystems and Diversity of the Sierra Madre Occidental
Ecosystems and Diversity of the Sierra Madre Occidental M. S. González-Elizondo, M. González-Elizondo, L. Ruacho González, I.L. Lopez Enriquez, F.I. Retana Rentería, and J.A. Tena Flores CIIDIR I.P.N. Unidad Durango, Mexico Abstract—The Sierra Madre Occidental (SMO) is the largest continuous ignimbrite plate on Earth. Despite its high biological and cultural diversity and enormous environmental and economical importance, it is yet not well known. We describe the vegetation and present a preliminary regionalization based on physio- graphic, climatic, and floristic criteria. A confluence of three main ecoregions in the area corresponds with three ecosystems: Temperate Sierras (Madrean), Semi-Arid Highlands (Madrean Xerophylous) and Tropical Dry Forests (Tropical). The Madrean region harbors five major vegetation types: pine forests, mixed conifer forests, pine-oak forests, oak forests and temperate mesophytic forests. The Madrean Xerophylous region has oak or pine-oak woodlands and evergreen juniper scrub with transitions toward the grassland and xerophylous scrub areas of the Mexican high plateau. The Tropical ecosystem, not discussed here, includes tropical deciduous forests and subtropical scrub. Besides fragmentation and deforestation resulting from anthropogenic activities, other dramatic changes are occurring in the SMO, including damage caused by bark beetles (Dendroctonus) in extensive areas, particularly in drought-stressed forests, as well as the expansion of chaparral and Dodoneaea scrub at the expense of temperate forest and woodlands. Comments on how these effects are being addressed are made. Introduction as megacenters of plant diversity: northern Sierra Madre Occidental and the Madrean Archipelago (Felger and others 1997) and the Upper The Sierra Madre Occidental (SMO) or Western Sierra Madre is the Mezquital River region (González-Elizondo 1997). -
Xerophytic Species Evaluated for Renewable Energy Resources1
Purchased by U.S. Department of Agriculture for Official Use Xerophytic Species Evaluated for Renewable Energy Resources 1 M. E. CARR,2 B. S. PHILLIPS,2 AND M. O. BAGBy3 Previously, the USDA Northern Regional Research Center has examined 600 plant species in 88 families for their multipurpose, energy-producing potential. About three-fourths ofthese species have been from central and southern Illinois, but only about 2% have been from arid or semiarid regions ofthe United States. For this report, 100 species collected from Arizona were evaluated, bringing the total number ofspecies evaluated at this Center to 700 in 96 families. Plant spec imens were analyzed for yields offractions referred to as "oil," "polyphenol," "hydrocarbon," and protein and were examinedfor botanical characteristics. Oil and hydrocarbon fractions of selected species were partially characterized. Ten species gave high yields ofoil and/or polyphenol. For example, Asclepias linaria yielded 8.7% oil (dl)', ash-free sample basis) + 11.7% polyphenol (1.9Q6 hydrocar bon). Rhus choriophylla yielded 7.0% oil + 20% polyphenol (0.4% hydrocarbon) and Juglans major yielded 7.0% oil + 9.4% polyphenol (0.2% hydrocarbon). Pit tosporum tobira gave the highest yield ofhydrocarbon (2.3%). Fourteen species contained at least 18% protein. In general, the percentages of species yielding substantial amounts of oil and/or polyphenol were considerably higher for the Arizona species than for those 600 species previously analyzed. Complete analyt ical data are presentedfor 38 species and are discussed in relationship to the 600 species previously reported. In recent years, there has been much interest in developing and using more effectively plants that are able to tolerate arid and semiarid areas, particularly for industrial nonfood uses (Davis et aI., 1983). -
Reference Plant List
APPENDIX J NATIVE & INVASIVE PLANT LIST The following tables capture the referenced plants, native and invasive species, found throughout this document. The Wildlife Action Plan Team elected to only use common names for plants to improve the readability, particular for the general reader. However, common names can create confusion for a variety of reasons. Common names can change from region-to-region; one common name can refer to more than one species; and common names have a way of changing over time. For example, there are two widespread species of greasewood in Nevada, and numerous species of sagebrush. In everyday conversation generic common names usually work well. But if you are considering management activities, landscape restoration or the habitat needs of a particular wildlife species, the need to differentiate between plant species and even subspecies suddenly takes on critical importance. This appendix provides the reader with a cross reference between the common plant names used in this document’s text, and the scientific names that link common names to the precise species to which writers referenced. With regards to invasive plants, all species listed under the Nevada Revised Statute 555 (NRS 555) as a “Noxious Weed” will be notated, within the larger table, as such. A noxious weed is a plant that has been designated by the state as a “species of plant which is, or is likely to be, detrimental or destructive and difficult to control or eradicate” (NRS 555.05). To assist the reader, we also included a separate table detailing the noxious weeds, category level (A, B, or C), and the typical habitats that these species invade. -
Tree Recommendations
New Mexico State University, Cooperative Extension Service www.aces.nmsu.edu Shrubs Vines Trees Palms Citrus ‘Landscape Trees in the Southwest’ Discovering the beautiful possibilities of what can be grown in our southwestern desert climates Washington D.C. Tree Canopies for Walkability of City Streets, Reduction of the Heat Island Effect Red Oaks Tree Groups to Consider Zelkova/Elm trees Fagaceae/Oak Family Heritage Seedlings and Liners, Salem, Oregon (heritageseedlings.com) ‘Mesa de Maya’ Oak Quercus grisea ‘Grey Oak’ Quercus muhlenbergii ‘Chinquapin Oak’ Quercus rugosa ‘Net Leaf Oak’ Quercus laceyi ‘Lacey Oak’ Quercus oblongifolia ‘Mexican Blue Oak’ Quercus robur x alba 'Crimschmidt‘ ‘CRIMSON SPIRE OAK’ Quercus albocicta ‘Cusi Oak’ Yécora, Sonora, Mexico Quercus germana ‘Mexican Royal Oak’ Ulmaceae/Elm Family Celtis reticulata ‘Net Leaf Hackberry’ Sapindaceae/Maple Family Sapindus drummondii ‘Western Soapberry’ Ungnadia speciosa ‘Mexican Buckeye’ Acer grandidentatum ‘Big Tooth Maple’ ‘Big Tooth Maple’ Acer grandidentatum ‘Mesa Glow’ NMSU, New Tree Introduction through J. Frank Schmidt & Son Company, Boring, OR Acer sempervirens ‘Cretan Maple’ Acer sempervirens ‘Cretan Maple’ Cashew/Anacardiaceae Family Pistacia chinensis Pistacia chinensis ‘Keith Davey’ Pistacia ‘Red Push’ Pistacia mexicana Pistacia lentiscus ‘Mastic Gum Tree’ Pistacia lentiscus Pistacia lentiscus Rhus ovata Rhus ovata Olive/Oleaceae Family Chionanthus retusus ‘Chinese Fringe Tree’ Chionanthus retusus ‘Chinese Fringe Tree’ Foresteria neomexicana Fraxinus greggii ‘Little -
SF Street Tree Species List 2019
Department of Public Works 2019 Recommended Street Tree Species List 1 Introduction The San Francisco Urban Forestry Council periodically reviews and updates this list of trees in collaboration with public and non-profit urban forestry stakeholders, including San Francisco Public Works, Bureau of Urban Forestry and Friends of the Urban Forest. The 2019 Street Tree List was approved by the Urban Forestry Council on October 22, 2019. This list is intended to be used for the public realm of streets and associated spaces and plazas that are generally under the jurisdiction of the Public Works. While the focus is on the streetscape, e.g., tree wells in the public sidewalks, the list makes accommodations for these other areas in the public realm, e.g., “Street Parks.” While this list recommends species that are known to do well in many locations in San Francisco, no tree is perfect for every potential tree planting location. This list should be used as a guideline for choosing which street tree to plant but should not be used without the help of an arborist or other tree professional. All street trees must be approved by Public Works before planting. Sections 1 and 2 of the list are focused on trees appropriate for sidewalk tree wells, and Section 3 is intended as a list of trees that have limited use cases and/or are being considered as street trees. Finally, new this year, Section 4, is intended to be a list of local native tree and arborescent shrub species that would be appropriate for those sites in the public realm that have more space than the sidewalk planting wells, for example, stairways, “Street Parks,” plazas, and sidewalk gardens, where more concrete has been extracted. -
Southwestern Rare and Endangered Plants: Proceedings of the Fourth Conference
Southwestern Rare and Endangered Plants : UnitedUnited States States DepartmentDepartment ofof Agriculture Agriculture ForestForest Service Service Proceedings of the Fourth RockyRocky Mountain Mountain ResearchResearch Station Station Conference ProceedingsProceedings RMRS-P-48CD RMRS-P-48CD JulyJuly 2007 2007 March 22-26, 2004 Las Cruces, New Mexico Barlow-Irick, P., J.J. AndersonAnderson andand C.C. McDonald,McDonald, techtech eds.eds. 2007.2006. SouthwesternSouthwestern rarerare andand endangered plants: Proceedings of the fourth conference; March 22-26, 2004; Las Cruces, New Mexico. Proceedings RMRS-P-XX.RMRS-P-48CD. Fort Fort Collins, Collins, CO: CO: U.S. U.S. Department of Agriculture, Forest Service,Service, Rocky Mountain ResearchResearch Station.Station. 135 pp.p. Abstract These contributed papers review the current status of plant conservation in the southwestern U.S. Key Words: plant conservation, conservation partnerships, endangered plants, plant taxonomy, genetics, demography, reproductive biology, biogeography, plant surveys, plant monitoring These manuscripts received technical and statistical review. Views expressed in each paper are those of the authors and not necessarily those of the sponsoring organizations or the USDA Forest Service. Cover illustration: Have Plant Press, Will Travel by Patricia Barlow-Irick You may order additional copies of this publication by sending your mailing information in label form through one of the following media. Please specify the publication title and series number. Fort Collins Service Center Telephone (970) 498-1392 FAX (970) 498-1122 E-mail [email protected] Web site http://www.fs.fed.us/rmrs Mailing address Publications Distribution Rocky Mountain Research Station 240 West Prospect Road Fort Collins, CO 80526 USDA Forest Service Proceedings RMRS-P-XXRMRS-P-48CD Southwestern Rare and Endangered Plants: Proceedings of the Fourth Conference March 22-26, 2004 Las Cruces, New Mexico Technical Coordinators: Patricia Barlow-Irick Largo Canyon School Counselor, NM John Anderson U.S.