Laser-Induced Excitation Mechanisms and Phase Transitions in ☆ Spectrochemical Analysis – Review of the Fundamentals
Total Page:16
File Type:pdf, Size:1020Kb
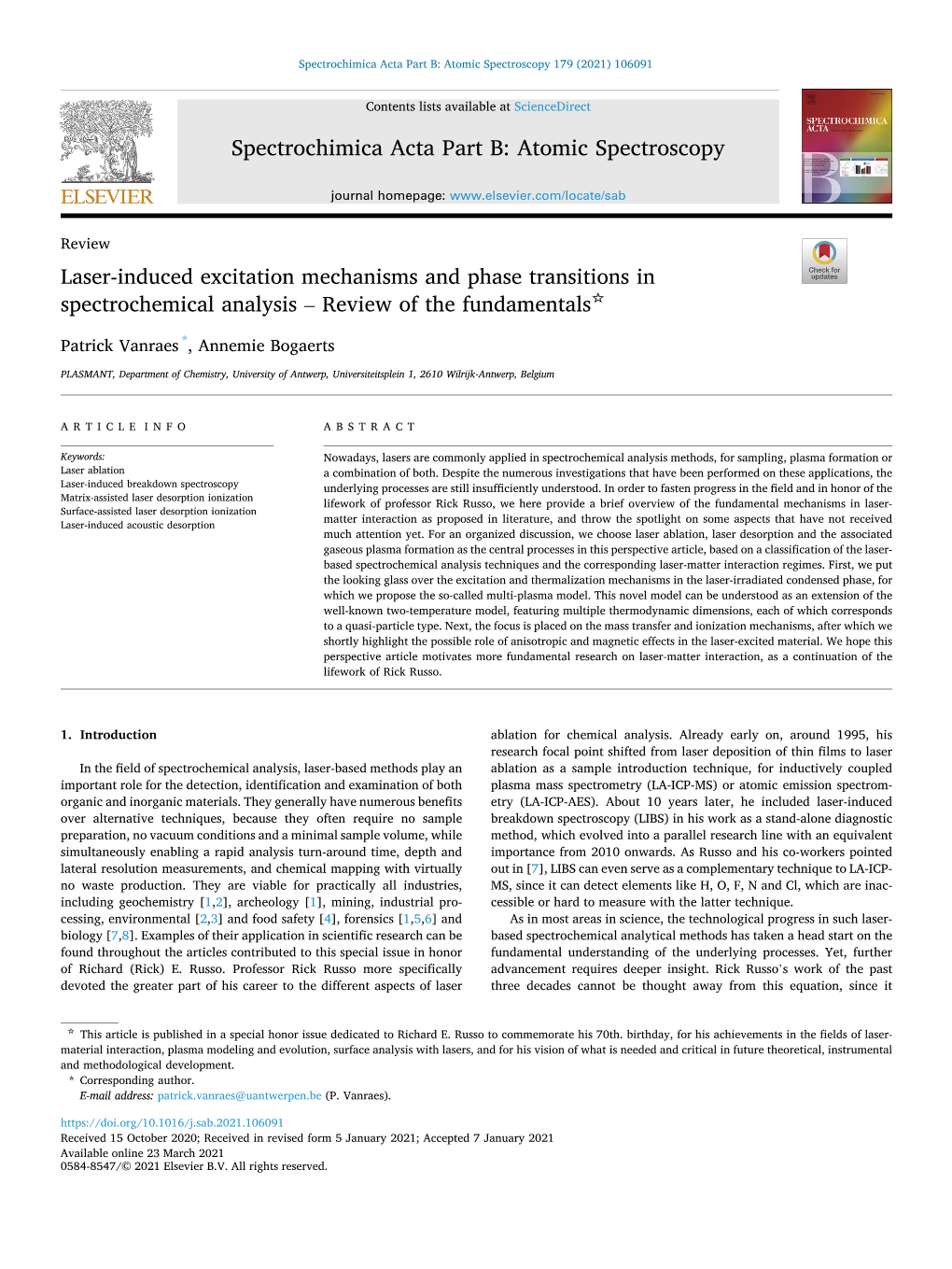
Load more
Recommended publications
-
Development and Characterization of an Atmospheric Pressure Ionization Source Matrix-Assisted Laser Desorption Electrospray Ioni
ABSTRACT SAMPSON, JASON SCOTT. Development and Characterization of an Atmospheric Pressure Ionization Source Matrix-Assisted Laser Desorption Electrospray Ionization Coupled to Fourier Transform Ion Cyclotron Resonance Mass Spectrometry for Analysis of Biological Macromolecules. (Under the direction of David Charles Muddiman). The field of mass spectrometry has grown tremendously over the past few decades due in large part to the continued application to new and interesting areas of exploration. The advent of soft ionization sources such as electrospray ionization and matrix-assisted laser desorption/ionization has dramatically increased the applications of mass spectrometry; in particular, analysis of complex biological samples. Electrospray ionization has demonstrated the capability to generate multiply-charged ions which increases the amount of information garnered from each analysis. Matrix-assisted laser desorption/ionization has demonstrated complex sample analysis requiring minimal sample preparation, which results in high throughput. As a result of these findings, there has been tremendous growth in the development of new ionization source technology in recent years for reducing sample preparation required prior to analysis for high throughput sample analysis and the generation of multiply-charged ions. Demonstrated herein is the development and characterization of an atmospheric pressure ionization source called matrix-assisted laser desorption electrospray ionization (MALDESI). MALDESI is a hybrid combination of MALDI and ESI which utilizes laser desorption with electrospray postionization for the generation of multiply-charged ions. Multiply-charged ions are of particular importance when using Fourier transform mass spectrometry, due to the increase in resolving power and mass accuracy with increasing charge on the molecule. Positive identification of biological macromolecules is demonstrated utilizing top-down characterization of intact polypeptides as well as high mass accuracy utilizing internal calibration. -
With Femtosecond Lasers, 572-74, 573/ Hydrodynamic Motion
Index Ablation Central hot spark ignition, by direct drive of ceramics, 216-21 implosion, 380-83 dielectric function during, 192-93, 193/ Central ignition scheme, 377/ 378 with femtosecond lasers, 572-74, 573/ Ceramics, ultrashort laser pulse interactions hydrodynamic motion role in, 2, 5-6 with, 216-21,217/218/ initial temperature at threshold of, 3 Coherent anti-Stokes Raman scattering measurement of dynamics of, 190, 191/ (CARS), 198, 206-8, 207/ of polymers, 225-26, 225/ 226/ 281-95 Coherent control of polymers with EUV laser, 535^0, 536/ raman spectroscopy, 206-8, 207/ 537/ 538/ 538<, 539/ of ultrashort laser pulse, 203^, 203/ spectral control of, 204-5, 205/ 206/ Collisional ionization, of dielectrics, 26-27 stages of, 68 69, 69/ Cosine model, of laser ionization, 110-14, Ablative cleaning, 37 110/115/ Ablative laser propulsion, 408-11, 409/ Coulomb explosion (CE) Artworks. See Painted artworks criterion for, 20-21, 21/ Asymptotic density profile, 12, 13/ features of, 18 from ultrashort laser pulse, 17-32 Band structure, 99 experimental evidence for, 18-20 Biological scaffold structures, from Crater ORMOCER®s, 149-51, 150/ 151/ formation of, 7-8, If Boltzmann plot, of plume, 77, 77/ hydrodynamic motion formation of, 4 Brillouin zone (BZ), of laser ionization, Crystal expansion, ultrashort laser pulse for, 100-108, 101/ 108/ 110 1-15 Bubble formation conclusion of, 14-15 calculation of, 254-57 hydrodynamic motion characteristics, 4—6 cavitation bubble dynamics, 262-67, 263/ hydrodynamic simulation, 11-14 264/ 265/ interference during, 9-11,10/ pulse trains with energies above threshold introduction to, 1-3 for, 271-72 shell and crater formation, 7-8, If pulse trains with energies below threshold for, 269-71,269/ Desorption ionization on silicon (DIOS) stress-induced, 254—67 MALDIv., 518-19, 518/521/ threshold for, 261-62 asSLDI, 506-7, 515-19 BZ. -
Surface‐Assisted Laser Desorption/Ionization Mass Spectrometry Imaging: a Review
Received: 27 July 2020 | Revised: 22 October 2020 | Accepted: 24 October 2020 DOI: 10.1002/mas.21670 REVIEW ARTICLE Surface‐assisted laser desorption/ionization mass spectrometry imaging: A review Wendy H. Müller | Alexandre Verdin | Edwin De Pauw | Cedric Malherbe | Gauthier Eppe Mass Spectrometry Laboratory, MolSys Research Unit, Chemistry Department, Abstract University of Liège, Liège, Belgium In the last decades, surface‐assisted laser desorption/ionization mass spectro- metry (SALDI‐MS) has attracted increasing interest due to its unique capabilities, Correspondence Gauthier Eppe, Mass Spectrometry achievable through the nanostructured substrates used to promote the analyte Laboratory, MolSys Research Unit, desorption/ionization. While the most widely recognized asset of SALDI‐MS is Chemistry Department, University of Liège, Allée du Six Août, 11—Quartier the untargeted analysis of small molecules, this technique also offers the possi- Agora, B‐4000 Liège, Belgium. bility of targeted approaches. In particular, the implementation of SALDI‐MS Email: [email protected] imaging (SALDI‐MSI), which is the focus of this review, opens up new oppor- tunities. After a brief discussion of the nomenclature and the fundamental me- chanisms associated with this technique, which are still highly controversial, the Acronyms: 9‐AA, 9‐aminoacridine; AgLDI, silver‐assisted laser desorption/ionization; AgNPET, silver nanoparticle‐enhanced target; AMP, adenosine monophosphate; ATP, adenosine triphosphate; AuNPET, gold nanoparticle‐enhanced target; BP, -
Tip-Enhanced Laser Ablation Sample Transfer for Mass Spectrometry
Louisiana State University LSU Digital Commons LSU Doctoral Dissertations Graduate School 2016 Tip-Enhanced Laser Ablation Sample Transfer for Mass Spectrometry Chinthaka Aravinda Seneviratne Louisiana State University and Agricultural and Mechanical College, [email protected] Follow this and additional works at: https://digitalcommons.lsu.edu/gradschool_dissertations Part of the Chemistry Commons Recommended Citation Seneviratne, Chinthaka Aravinda, "Tip-Enhanced Laser Ablation Sample Transfer for Mass Spectrometry" (2016). LSU Doctoral Dissertations. 2355. https://digitalcommons.lsu.edu/gradschool_dissertations/2355 This Dissertation is brought to you for free and open access by the Graduate School at LSU Digital Commons. It has been accepted for inclusion in LSU Doctoral Dissertations by an authorized graduate school editor of LSU Digital Commons. For more information, please [email protected]. TIP-ENHANCED LASER ABLATION SAMPLE TRANSFER FOR MASS SPECTROMETRY A Dissertation Submitted to the Graduate Faculty of the Louisiana State University and Agricultural and Mechanical College in partial fulfillment of the requirements for the degree of Doctor of Philosophy in The Department of Chemistry by Chinthaka Aravinda Seneviratne M.S., Bucknell University, 2011 B.Sc., University of Kelaniya, 2008 May 2016 This dissertation is dedicated with love to my parents, Hector and Dayani Seneviratne my wife Sameera Herath and my little buddy Julian !. ii ACKNOWLEDGEMENTS First and foremost, I give glory to God. Without God’s blessings, none of this would have been possible. It is a great pleasure to offer my unbounding appreciation for all who have supported and guided me over the course my doctoral studies to the completion of this dissertation. I would like to thank my doctoral mentor, Dr. -
Chapter 20 SOFT LASER DESORPTION IONIZATION - MALDI, DIOS and NANOSTRUCTURES
Chapter 20 SOFT LASER DESORPTION IONIZATION - MALDI, DIOS AND NANOSTRUCTURES Akos Veites Department of Chemistry, Institute for Proteomics Technology and Applications, The George Washington University, Washington DC 20052 1. INTRODUCTION In 1948, Ame Tiselius won the Nobel Prize in chemistry for the discovery of electrophoresis and its application to analyze large molecules, specifically complex serum proteins. During the following decades, gel electrophoresis and its derivative techniques became a central method for molecular biology. They allowed for the identification of molecular mass with an accuracy of ~1 kDa, i.e., a thousand atomic mass units. Although this method enabled the separation of thousands of proteins from biological samples, the determination of the actual molecular weight, and with it finding the true identity of many substances, remained unachievable. When it came to accurate mass determination of small molecules, mass spectrometry was the method of choice. However, for the accurate mass analysis of large molecules, their low volatility presented a fundamental obstacle. Typically, the larger the molecule, the harder it is to transfer it into the gas phase without degradation. Depositing the energy necessary for volatilization initiates other competing processes that elevate the internal energy of the adsorbate and results in its fragmentation. This competition between evaporation (or desorption) and fragmentation was recognized early on and the method of rapid heating was proposed to minimize the latter (Beuhler, et al., 1974). Lasers with submicrosecond pulse length were appealing tools for rapid heating but the initial results indicated limitations with respect to the ultimate size of the biomolecules (m/z < 1,500) that could 506 Laser Ablation and its Applications be successfully analyzed (Posthumus ,et al., 1978). -
Synthesis of Platinum Nanoparticles and the Study of Their Efficiency As Substrates for Laser Desorption/Ionization (LDI) of Model Analytes
Platinum nanoparticles synthesized by laser ablation in water and their use as substrates for the soft laser desorption/ionization of polymers and peptides Maite Cueto1*, F. Gámez2, A. R. Hortal2, P. Hurtado2, B. Martínez-Haya2 M. Sanz3, M. Oujja3, M. Castillejo3 1 Instituto de Estructura de la Materia CSIC, Madrid (Spain) 2 Departamento de Sistemas Físicos, Químicos y Naturales, Universidad Pablo de Olavide, 41013 Seville (Spain) 3 Instituto de Química Física Rocasolano CSIC , Madrid (Spain) *E-mail: [email protected] Summary - The object of this work is the synthesis of platinum nanoparticles and the study of their efficiency as substrates for laser desorption/ionization (LDI) of model analytes. - Platinum stands out among the noble metals as a nanostructured assisted LDI (NALDI) active substrate because of its low heat conductivity and high melting temperature [1]. - We have synthesized Platinum nanoparticles by high energy pulsed laser ablation of Pt foil in aqueous solution. - Different Nd:YAG laser wavelengths (266, 532, 1064 nm) and stabilizing agents (PEG, PVA, citrate) have been used to control the nanoparticle size and crystallinity [2,3]. - Pt nanoparticles with single and bimodal size distributions (ranging 2-30 nm) and of spherical and rod-like shape have been obtained. - The nanoparticles have been tested as active substrates for the soft laser desorption/ionization (NALDI-MS) of a synthetic polymer (polyethylenglycol PEG600) and a peptide (Angiotensin I). - The NALDI-MS experiments were performed in positive-ion mode with a commercial time-of-flight mass spectrometer (UltrafleXtreme, Bruker, 355nm laser source) and demonstrate that Platinum nanoparticles provide a good sensitivity for the detection of these model analytes. -
Infrared Laser Desorption/Ionization Mass Spectrometry
Louisiana State University LSU Digital Commons LSU Doctoral Dissertations Graduate School 2006 Infrared laser desorption/ionization mass spectrometry: fundamental and applications Mark Little Louisiana State University and Agricultural and Mechanical College, [email protected] Follow this and additional works at: https://digitalcommons.lsu.edu/gradschool_dissertations Part of the Chemistry Commons Recommended Citation Little, Mark, "Infrared laser desorption/ionization mass spectrometry: fundamental and applications" (2006). LSU Doctoral Dissertations. 2690. https://digitalcommons.lsu.edu/gradschool_dissertations/2690 This Dissertation is brought to you for free and open access by the Graduate School at LSU Digital Commons. It has been accepted for inclusion in LSU Doctoral Dissertations by an authorized graduate school editor of LSU Digital Commons. For more information, please [email protected]. INFRARED LASER DESORPTION/IONIZATION MASS SPECTROMETRY: FUNDAMENTALS AND APPLICATIONS A Dissertation Submitted to the Graduate Faculty of the Louisiana State University and Agricultural and Mechanical College in partial fulfillment of the requirements for the degree of Doctor of Philosophy in The Department of Chemistry by Mark Little B.S., Wake Forest University, 1998 December 2006 ACKNOWLEDGMENTS The popular quote, "L'enfer, c'est les autres" from an existential play by Jean-Paul Sartre is translated “Hell is other people”. I chose this quote because if it were not for all the “other people” in my life, I would be sitting on a beach right now sipping margaritas but I would not have my Ph.D. Therefore, I raise my salt covered glass to all my friends, family and colleagues for without their support this program of study would never been completed. -
Surface Modification and Laser Pulse Length Effects on Internal Energy Transfer in DIOS
24450 J. Phys. Chem. B 2005, 109, 24450-24456 Surface Modification and Laser Pulse Length Effects on Internal Energy Transfer in DIOS Guanghong Luo,† Yong Chen,† Gary Siuzdak,‡ and Akos Vertes*,† Department of Chemistry, Institute for Proteomics Technology and Applications, The George Washington UniVersity, Washington, D.C. 20052, and Center for Mass Spectrometry, The Department of Molecular Biology, The Scripps Research Institute, La Jolla, California 92037 ReceiVed: August 3, 2005; In Final Form: October 5, 2005 Benzyl-substituted benzylpyridinium (BP) chloride salts were used as a source of thermometer ions to probe the internal energy (IE) transfer in desorption/ionization on porous silicon (DIOS). To modify their wetting properties and the interaction energies with the thermometer ions, the DIOS surfaces were silylated to produce trimethylsilyl- (TMS), amine- (NH2), perfluoroalkyl- (PFA), and perfluorophenyl-derivatized (PFP) surfaces. Two laser sourcessa nitrogen laser with pulse length of 4 ns and a mode locked 3 × ω Nd:YAG laser with a pulse length of 22 psswere utilized to induce desorption/ionization and fragmentation at various laser fluence levels. The corresponding survival yields were determined as indicators of the IE transfer and the IE distributions were extracted. In most cases, with increasing the laser fluence in a broad range (∼20 mJ/cm2), no change in IE transfer was observed. For ns excitation, this was in remarkable contrast with MALDI, where increasing the laser fluence resulted in sharply (within ∼5 mJ/cm2) declining survival yields. Derivatization of the porous silicon surface did not affect the survival yields significantly but had a discernible effect on the threshold fluence for ion production. -
April 23-26, 2006
FOREWORD This abstract booklet provides a record of the 2006 U.S. Department of Energy (DOE) Analysis Contractors’ Meeting. The objective of this meeting is to provide a fruitful environment in which researchers with common interests will present and exchange information about their activities, will build collaborations among research groups with mutually reinforcing strengths, will identify needs of the research community, and will focus on opportunities for future research directions. The agenda has keynote talks, oral presentations, and posters; with ample time for discussion and interactions, we emphasize that this is an informal meeting for exchange of information and building of collaborations; it is not a review of researchers’ achievements or a forum to choose future directions. We are pleased to have the privilege of organizing this joint meeting and of serving as the managers for the research program. In carrying out these tasks, we learn from the achievements and share the excitement of the research of the many sponsored scientists and students whose names appear on the papers in the following pages. We also hope that this meeting will enhance your research efforts and will nurture future collaborations and initiatives. We thank all of the researchers whose dedication and innovation have advanced our research and made this meeting possible and, we hope, productive. We hope that all of you will build on your successes and that we will assemble in a very few years for our next meeting. We thank Diane Marceau of the Chemical Sciences, Geosciences and Biosciences Division, and Sophia Kitts and Angie Lester of the Oak Ridge Institute for Science and Education for the technical and logistical features of this meeting. -
Li Et Al. (2017)
International Journal of Mass Spectrometry 422 (2017) 177–187 Contents lists available at ScienceDirect International Journal of Mass Spectrometry jou rnal homepage: www.elsevier.com/locate/ijms Young Scientist Feature Mars Organic Molecule Analyzer (MOMA) laser desorption/ionization source design and performance characterization a,∗ b a c d Xiang Li , Ryan M. Danell , Veronica T. Pinnick , Andrej Grubisic , Friso van Amerom , e e e,∗ Ricardo D. Arevalo Jr. , Stephanie A. Getty , William B. Brinckerhoff , f e g Adrian E. Southard , Zachary D. Gonnsen , Tomoko Adachi a Center for Space Science & Technology, University of Maryland, Baltimore County, Baltimore, MD, USA b Danell Consulting, Inc., Winterville, NC, USA c Center for Research and Exploration in Space Science & Technology, University of Maryland, College Park, College Park, MD, USA d Mini-Mass Consulting, Inc., Hyattsville, MD, USA e NASA Goddard Space Flight Center, Greenbelt, MD, USA f Universities Space Research Association, Columbia, MD, USA g Catholic University, Washington DC, USA a r t i c l e i n f o a b s t r a c t Article history: The Mars Organic Molecule Analyzer (MOMA), a dual-source, ion trap-based instrument capable of both Received 6 August 2016 pyrolysis-gas chromatography mass spectrometry (pyr/GC–MS) and laser desorption/ionization mass Received in revised form 26 January 2017 spectrometry (LDI-MS), is the core astrobiology investigation on the ExoMars rover. The MOMA instru- Accepted 28 March 2017 ment will be the first spaceflight mass analyzer to exploit the LDI technique to detect refractory organic Available online 21 May 2017 compounds and characterize host mineralogy; this mode of analysis will be conducted at Mars ambient conditions. -
Advanced Information on the Nobel Prize in Chemistry 2002, 9 October 2002
Advanced information on the Nobel Prize in Chemistry 2002, 9 October 2002 Information Department, P.O. Box 50005, SE-104 05 Stockholm, Sweden Phone: +46 8 673 95 00, Fax: +46 8 15 56 70, E-mail: [email protected], Website: www.kva.se Mass spectrometry (MS) and nuclear magnetic resonance (NMR) applied to biological macromolecules The Nobel Prize in Chemistry for 2002 is to be shared between scientists working on two very important methods of chemical analysis applied to biological macromolecules: mass spectrometry (MS) and nuclear magnetic reso- nance (NMR). Laureates John B. Fenn, Koichi Tanaka (MS) and Kurt Wüthrich (NMR) have pioneered the successful application of their techniques to biological macromolecules. Biological macromolecules are the main actors in the makeup of life whether expressed in prospering diversity or in threatening disease. To understand biology and medicine at molecular level where the identity, functional cha- racteristics, structural architecture and specifc interactions of biomolecules are the basis of life, we need to visua- lize the activity and interplay of large macromolecules such as proteins. To study, or analyse, the protein molecules, principles for their separation and determination of their individual characteristics had to be developed. Two of the most important chemical techniques used today for the analysis of biomolecules are mass spectrometry (MS) and nuclear magnetic resonance (NMR), the subjects of this year’s Nobel Prize award. Mass spectrometry (MS) The ability to separate molecules based on different size and charge was frst described in 1912 by J.J. Thompson (Nobel Prize laureate in 1906 for investigations of the conduction of electricity by gases) and expressed as the mass/charge ratio with the unit Thompson (Th). -
Élelmiszervizsgálati Közlemények Journal of Food Investigation
ÉLELMISZERVIZSGÁLATI KÖZLEMÉNYEK JOURNAL OF FOOD INVESTIGATION TUDOMÁNY-ÉLET-minősÉG-BIZTONSÁG LX. ÉVFOLYAM 4. SZÁM 2014. DECEMBER 31. Tömegspektrometria a mikrobiológiában Mass-spectrometry in microbiology Előrejelző mikrobiológia Búcsú Farkas József akadémikustól Aszpartám az élelmiszerekben Hársmézminták jellemzői Magmérő eljárások búzánál Hatósági terméktesztek a Szupermenta blogon A fűszerpaprika jogi szabályozása Csomagolóanyagok uniós követelményei Predictive microbiology • Aspartame in foods • Parameters of linden honey samples • Wheat kernel measurement techniques • Authority product tests on Supermenta blog • Legal regulation of paprika • EU-requirements of food con- tact materials www.eviko.hu Kedves Olvasóink! TARTalom – ConTENTS Dear Readers, Alig telt el egy esztendő, amióta tele lendülettel, It was not quite a year ago that we set out to rejuvenate our bizalommal, tenni akarással nekirugaszkodtunk journal, full of energy, hope and a willingness to work. It is with folyóiratunk megfiatalításának. Nehéz szívvel T Előrejelző mikrobiológiai modellezés, a kvantitatív mikrobiológiai 316 a heavy heart that I launch the fourth, year-end issue of Volume bocsátom útjára a megújult ÉVIK 60. évfolyam 60 of the renewed Journal of Food Investigations, while at the AR kockázatbecslés eszköze ( Farkas József , Mohácsiné Farkas Csilla) negyedik, év végi számát, hiszen most, Advent time of Advent, full of grace, we are one fewer. The eternal, Predictive microbiological modeling, a tool of quantitative microbiological kegyelemmel teli időszakában eggyel keveseb- honorary chairman of our editorial board, academician Prof. ben lettünk. Szerkesztőbizottságunk örökös, Dr. József Farkas is no longer with us. He is remembered on T risk assessment ( József Farkas , Csilla Mohácsiné Farkas) tiszteletbeli elnöke, Prof. Dr. Farkas József the pages of our year-end issue. He who burnt the candle of ALOM akadémikus már nincs közöttünk.