Eo-Alpine Evolution of the Central Southern Alps Insights from Structural Analysis and New Geochronological Constraints
Total Page:16
File Type:pdf, Size:1020Kb
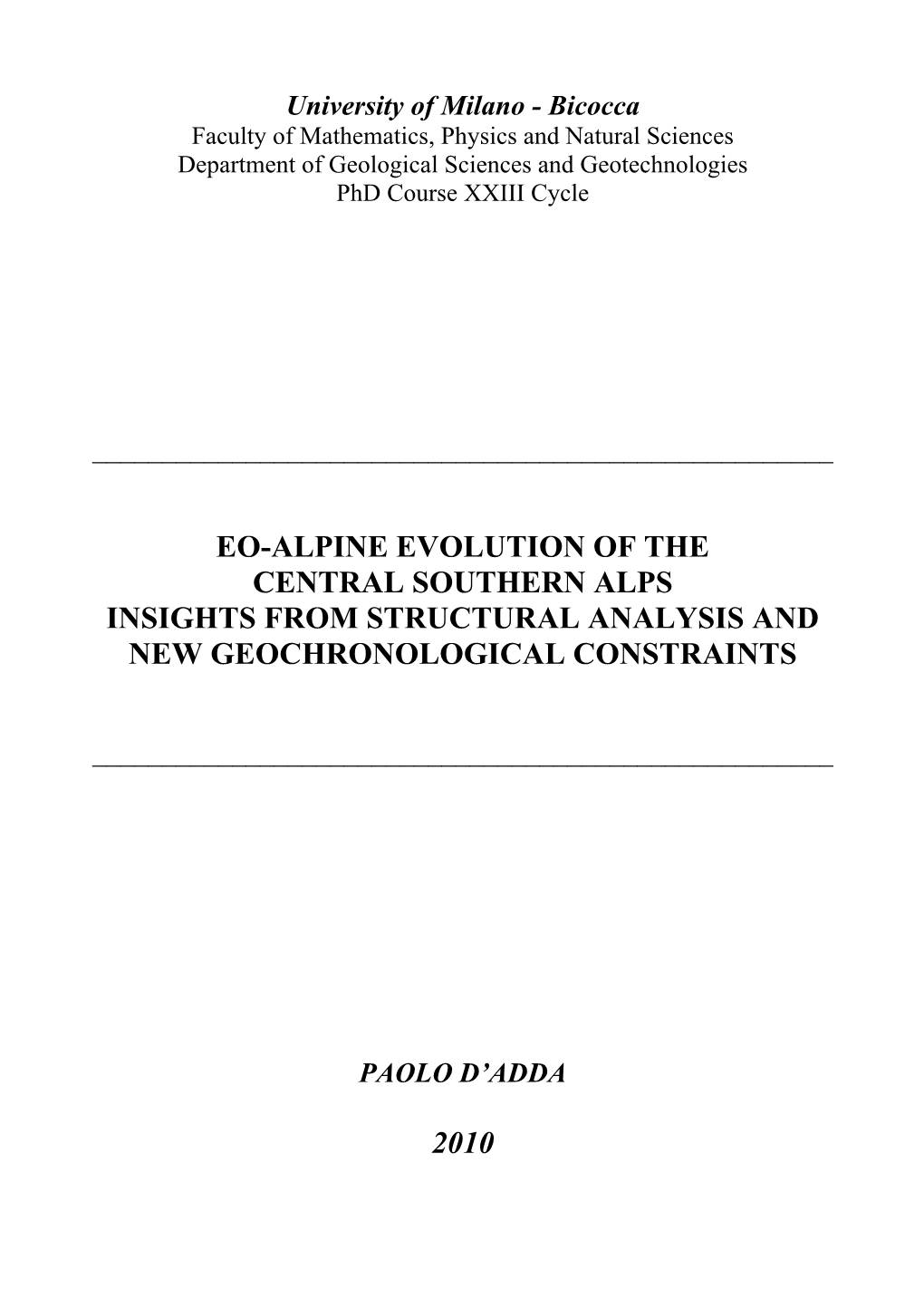
Load more
Recommended publications
-
ALLEGATO 4: CARTOGRAFIA DI INQUADRAMENTO (Fonte: Sistema Informativo Territoriale – SITER - Della Provincia Di Bergamo)
ALLEGATO 4: CARTOGRAFIA DI INQUADRAMENTO (fonte: Sistema Informativo TERritoriale – SITER - della Provincia di Bergamo) VILMINORE DI SCALVE VALGOGLIO GROMO COLERE ´ OLTRESSENDA ALTA ARDESIO ANGOLO TERME CASTIONE DELLA PRESOLANA FINO DEL MONTE VILLA D`OGNA PARRE PIARIO ONORE CLUSONE ROGNO SONGAVAZZO CERETE ROVETTA COSTA VOLPINO BOSSICO SOVERE GANDINO LOVERE 1:50.000 Confine comunale Industriale Raffreddamento Scarico di emergenza privato Scarico depurato pubblico Terminale pubblica fognatura bianche Scarico di emergenza (Staz. sol. / bypass) Sfioratore Sfioratore/scarico staz. sollevamento Reticolo idrografico Carta degli scarichi autorizzati in corpo d'acqua superficiale VILMINORE DI SCALVE VALGOGLIO GROMO COLERE ´ OLTRESSENDA ALTA ARDESIO ANGOLO TERME CASTIONE DELLA PRESOLANA FINO DEL MONTE VILLA D`OGNA PARRE PIARIO ONORE CLUSONE ROGNO SONGAVAZZO CERETE ROVETTA COSTA VOLPINO BOSSICO SOVERE GANDINO LOVERE 1:50.000 Confine comunale Sorgenti / Fontanili Derivazioni superficiali Pozzi Potabile Potabile Potabile Antincendio Antincendio Antincendio Igienico Igienico Igienico Industriale Industriale Industriale Produzione Energia Produzione Energia Produzione Energia Piscicoltura Piscicoltura Piscicoltura Zootecnico Zootecnico Irriguo Zootecnico Irriguo Uso Domestico Irriguo Uso Domestico Altro uso Uso Domestico Altro uso Altro uso Carta delle piccole derivazioni di acqua VILMINORE DI SCALVE VALGOGLIO GROMO COLERE ´ OLTRESSENDA ALTA ARDESIO ANGOLO TERME CASTIONE DELLA PRESOLANA FINO DEL MONTE VILLA D`OGNA PARRE PIARIO ONORE CLUSONE ROGNO SONGAVAZZO -
THE LEGENDARY LAKES of ITALY 2020 LAKES GARDA, COMO & MAGGIORE SELF-GUIDED CYCLE TOUR 280 Kms: 8 DAYS / 7 NIGHTS
THE LEGENDARY LAKES OF ITALY 2020 LAKES GARDA, COMO & MAGGIORE SELF-GUIDED CYCLE TOUR 280 kms: 8 DAYS / 7 NIGHTS One of Italy’s largest regions, Lombardy lies in the north of the country sharing a border with Switzerland. Stretching from the Alps to the lowlands of the Po Valley, it is home to a wide variety of stunning landscapes, including the breathtaking mountain chain that boasts the Valchiavenna, Valtellina and Camonica Valleys. The legendary beauty of these great lakes draws visitors to Sirmione and other well -known destinations dotted along the west side of Lake Garda, while Lake Como and Lake Maggiore are surrounded by stately homes, wild woodlands, picturesque park, chic towns and sleepy villages. The region is also characterised by the great flat tracts of the Po Valley lowlands with its shimmering mirrors of water. This is a destination that is varied, challenging and beautiful - perfect for a cycling tour. ITINERARY Day1: Arrival into Peschiera del Garda Peschiera del Garda is located at the southern end of Lake Garda, in Italy's Veneto region. The town's sixteenth-century island fortress and walls are visible today, a reminder of a time when Austria controlled the region. After discovering Peschiera's history, relax with a glass of Lugana, a local white wine. Day 2: Peschiera del Garda – Brescia Cycling 45 or 70 km From the harbour-fortress of Peschiera on Lake Garda, we descend upon the Mincio River and arrive at Monzambano, a town with a landmark castle above it. The peaceful rolling morainic hills accompany us to the enchantment of Castellaro Lagusello, surrounded by a medieval wall. -
COMUNE DI GANDINO Piazza V.Veneto N
studio associato Via Giorgio e Guido Paglia, n° 21 – 24122 BERGAMO – e-mail: [email protected] Tel. +39 035 248689 – +39 035 271216 – Fax +39 035 271216 REL.13-SS 11/10/2011 COMUNE DI GANDINO Piazza V.Veneto n. 7 – Gandino (Bg) PIANO URBANO GENERALE DEI SERVIZI NEL SOTTOSUOLO ai sensi del Regolamento Regionale n.6 del 15/02/2010 Relazione tecnica Bergamo, ottobre 2011 Sommario 1 FUNZIONE E TERMINI PER LA REDAZIONE DEL PUGSS.................................................................... - 4 - 2 DOCUMENTI DI RIFERIMENTO NELLA REDAZIONE DEL PUGSS....................................................... - 5 - 2.1 Riferimenti normativi......................................................................................................................... - 6 - 3 FASI REDAZIONALI ................................................................................................................................. - 8 - 3.1 Analisi metodologica......................................................................................................................... - 9 - 4 ELEMENTI COSTITUTIVI DEL PUGSS E RELATIVI CONTENUTI ....................................................... - 12 - 4.1 Rapporto territoriale........................................................................................................................ - 12 - 4.1.1 Sistema geoterritoriale............................................................................................................... - 12 - 4.1.2 Inquadramento geomorfologico e geologico............................................................................. -
Benedetta Gente La Mamma Di Riccardo E Quella Farfalla Che Le
PER TUTTO IL MESE DI LUGLIO ACQUISTANDO UNA CUCINA LUCE O CREO KITCHEN AVRAI IN OMAGGIO UN TELEVISORE 55” SAMSUNG VAL SERIANA, VAL DI SCALVE, ALTO E BASSO SEBINO, VAL CALEPIO, VAL CAVALLINA, BERGAMO APERTO DOMENICA POMERIGGIO Autorizzazione Tribunale di Bergamo: Quindicinale Pubblicità «Araberara» VENITE A TROVARCI! Numero 8 del 3 aprile 1987 NIARDO (BS) Tel. 0346/28114 Fax 0346/921252 Redazione Via S. Lucio, 37/24 - 24023 Clusone 26 luglio 2019 Composizione: Araberara - Clusone Tel. 0346/25949 Fax 0346/921252 “Poste italiane Spa - Spedizione in A.P. - D.L. 353/2003 Anno XXXIII - n. 14 (585) - € 2,00 Stampa: C.P.Z. Costa di Mezzate (Bg) (conv. in L. 27/02/2004 n° 46) art.1, comma 1, DCB Bergamo” Direttore responsabile: Piero Bonicelli CODICE ISSN 1723 - 1884 IL MARE RANZANICO A ROVESCIO Benedetta gente ARISTEA CANINI La mia immaginazione è di Piero Bonicelli limitata solo dalla mia im- maginazione. Quindi fac- Si vive la breve e bella estate da... villeggianti in “casa cio come voglio e ti imma- propria”, stranieri in terre che erano nostre e che non gino come mi piace, anche riconosciamo, frugando nel sottobosco alla ricerca di se non ti ho mai visto ma è radici (sociali e culturali) che non ricordiamo più dove l’ultima cosa che importa. cavolo le aveva piantate chi ci ha preceduto. Come il Oggi è il compleanno del vecchio becchino del mio paese che solo lui sapeva dov’e- Jennifer e Dario, mio papà, un compleanno ra sepolta la gente, al tempo in cui non c’erano le lapidi tondo, quindi lo festeggio, e solo le croci della vita e della morte e, quando è morto i due ‘novelli’ e non importa se non l’ho lui, nessuno sapeva più dove erano sepolti i parenti. -
SULZANO " Unending Dialogues Between Land and Islands" SULZANO GUIDE 3
TOURIST GUIDE SULZANO " unending dialogues between land and islands" SULZANO GUIDE 3 SULZANO BACKGROUND HISTORY much quicker and soon small Sulzano derives its name from workshops were turned into actual Sulcius or Saltius. It is located in an factories providing a great deal of area where there was once an ancient employment. Roman settlement and was born as a lake port for the area of Martignago. Along with the economic growth came wealth and during the Twentieth Once, the fishermen’s houses were century the town also became a dotted along the shore and around tourist centre with new hotels and the dock from where the boats would beach resorts. Many were the noble leave to bring the agricultural goods to and middle-class families, from the market at Iseo and the materials Brescia and also from other areas, from the stone quarry of Montecolo, who chose Sulzano as a much-loved used for the production of cement, holiday destination and readily built would transit through here directed elegant lake-front villas. towards the Camonica Valley. At the beginning of the XVI century, the parish was moved to Sulzano and thus the lake town became more important and bigger than the hillside Sulzano is a village that overlooks the one. During the XVII Century, many Brescia side of the Iseo Lake mills were built to make the most of COMUNE DI the driving force of the water that SULZANO flowed abundantly along the valley to the south of the parish. Via Cesare Battisti 91- Sulzano (Bs) Tel. 030/985141 - Fax. -
ELENCO Disponibilità Iniziali I Grado Per Operazioni Annuali 2019 20 Al 23 Agosto 2019
CLASSE DI CONCORSO A001 - Arte e Immagine CATTEDRE CATTEDRE ORE ANNUALI TEMPORANEE NOTE SCUOLE MEDIE RESIDUE (al 31/08/2020) (al 30/06/2020) BG "Corridoni" BGMM80701L BG "S.Lucia" BGMM80801C 1* C.O.E. BG "I Mille" BGMM8AF01B 6 BG "Muzio" BGMM811018 BG "Mazzi" BGMM812014 BG "Donadoni" BGMM81301X BG "A.da Rosciate" BGMM81401Q 6 BG "Camozzi" BGMM81501G 1 14 BG "Petteni" BGMM81601B 6 ALBANO S.A. BGMM817017 6 ALBINO BGMM818013 1 ALMENNO S.B. BGMM81901V 14 ALMENNO S.S. BGMM820013 2 ALZANO L.DO BGMM82101V 16 ARCENE BGMM82201P 12 AZZANO S.PAOLO BGMM82302G 2 BAGNATICA BGMM82401A 6 BARIANO BGMM825016 8 BONATE SOPRA BGMM826012 4 BONATE SOTTO BGMM82701T 12 BORGO DI TERZO BGMM82801N 14 BREMBATE SOPRA BGMM89501C 6 BREMBATE SOTTO BGMM82901D 1 6 BREMBILLA BGMM83001N 6 CALCINATE BGMM83101D CALCIO BGMM832019 CALUSCO D'ADDA BGMM833015 CAPRIATE S. G. BGMM834011 14 CARAVAGGIO BGMM83501R 16 CARVICO BGMM83601L 6 CASIRATE BGMM83702D 1 8 CASTELLI CALEPIO BGMM838018 8 CASAZZA BGMM839014 14 CHIUDUNO BGMM840018 2 CISANO B.SCO BGMM841014 1 6 CISERANO BGMM84201X 12 CLUSONE BGMM80601R 16 COLOGNO AL SERIO BGMM89901Q 10 COSTA VOLPINO BGMM84301Q 8 COVO BGMM84401G 18 CURNO BGMM84501B 6 DALMINE "Moro" BGMM8AB014 DALMINE "Camozzi" BGMM8AC01X 6 FARA GERA D'ADDA BGMM846028 4 GANDINO BGMM847024 4 GAZZANIGA BGMM84801V 10 GORLAGO "A.Moro" BGMM84901P 2 GORLE BGMM85001V GROMO BGMM85101P 6 GRUMELLO BGMM85202G 20 LEFFE BGMM85301A OSIO SOPRA BGMM854016 10 LOVERE BGMM855012 16 LOVERE CONVITTO BGMM89401L CLASSE DI CONCORSO A001 - Arte e Immagine CATTEDRE CATTEDRE ORE ANNUALI TEMPORANEE -
MERCOLEDI' 13 DICEMBRE 2017 Cirano (Gandino
CLUB ALPINO ITALIANO SEZIONE DI BERGAMO “Antonio Locatelli” -1873- tel. 035-4175475 www.caibergamo.it Gruppo Seniores “E. Bottazzi” MERCOLEDI’ 13 DICEMBRE 2017 Cirano (Gandino) – Rif. Malga Longa -A monte dell’abitato di Gandino, compresa nella frazione di Cirano, si trova la cinquecentesca chiesa dei santi Gottardo e Bartolomeo Apostolo. Il piccolo campanile ospita un concerto di 9 campane in Do 4. La Campana maggiore è antica, fusa nel 1650. -La Malga Lunga, situata in posizione dominante sui monti tra Sovere e Gandino, è una roccaforti partigiane del periodo resistenziale. Nel 1944 vi si insedia la 53ma Brigata Garibaldi che assume il nome “Tredici Martiri di Lovere” in onore dei tredici partigiani fucilati a Lovere il 22 dicembre 1943. H Disl.Sal. Lung. Totale h Difficoltà Fatica Durata gg Tipo gita max.m m Km A/R 1383 988 15 6 E F1/F2 1 Esc scarponi bastoncini abbigl. montagna altre attrezzature XX X X Parapioggia/Ramponcini PARTENZA CON PROPRIE AUTO DALLO STADIO ALLE ORE 07:00. Direzione Gandino con uscita Fiorano, sulla statale della Val Seriana. Superato il centro di Gandino proseguire per la frazione di Cirano (728 m) in Valle Concossolo. Parcheggiato le macchine nel parcheggio di Piazza Mons. Giovanni Antonietti vicino alla chiesa, si prosegue sul sentiero CAI 548 in direzione San Gottardo (625 m). Si prose ancora sul sentiero 548 fino alla bella spianata di Campo d’Avene (1267 m). Costellata da numerose e caratteristiche doline, con una grande cascina al centro dello spettacolare pascolo. Da questa posizione sulla mulattiera CAI 545 si prosegue direttamente alla Malga Longa, oppure la facoltà di salire alla Baita di Monte Alto (1383 m) e poi riscendere per riprendere il cammino sul CAI 545, proveniente dal Campo d’Avene. -
Giro D'italia 2021
Anno 2021 www.valtellina.it Sentiero Valtellina Giro d’Italia e Ciclabile Valchiavenna: 10 luoghi da non perdere 2021 Sentiero Valtellina and Ciclabile Valchiavenna: 10 must-see places I passi alpini chiudono al traffico The alpine passes are closed to all traffic Mountain bike che passione! Mountain bike, what a passion! Mondo E-bike E-bike world v Foto di copertina: Strada per il Mortirolo, versante di Mazzo www.valtellina.it | 3 Benvenuto in Valtellina! Passo del Gallo Coira Coira Zurigo Zurigo Passo S. Maria Merano Livigno Bolzano Passo Spluga Valdidentro St. Moritz Passo Stelvio SVIZZERA Bormio Madesimo Passo Bernina Passo Forcola Valfurva v Pista Stelvio, Bormio Valdisotto S. Caterina TRENTINO Valfurva Passo Maloja ALTO ADIGE Chiavenna Chiesa in Sondalo Passo Gavia Valchiavenna Valmalenco Grosio Passo Mortirolo Trento Valmalenco Ponte di v Snowpark Mottolino, Livingo Valmasino Legno Teglio Tirano Morbegno Edolo Aprica Sondrio Passo Val Tartano dell’Aprica Valli del Bitto Passo S. Marco Lago di Como LOMBARDIA Lecco Como Paesaggi e natura accompagnati da buon vino, piatti tipici, This is a land of unspoiled nature, fine wine, unique cuisine luoghi di cultura e paradisi termali. Questa è la Valtellina: and culture, and thermal wonderlands. This is Valtellina: una regione interamente montana situata a nord della a region made up entirely of mountains in the north Verso Milano-Cortina 2026… Lombardia, al confine tra l’Italia e il Cantone svizzero of Lombardy, on the border between Italy and Swiss dei Grigioni, nel cuore delle Alpi. canton -
Curriculum Vitae Di
Curriculum vitae di Lorenzo Ferrari Dott. Ing. Lorenzo Ferrari Via Cornasola n.8/a – 24065 Lovere (Bergamo) Curriculum vitae di Lorenzo Ferrari Gennaio – Maggio 2004 Esperienze professionali - Inizio collaborazione presso Agenzia Immobiliare Atlas s.a.s. di Darfo B.T. (Bs) [REF 3]. Giugno 2004 - Iscrizione nel registro dei praticanti geometri della provincia di Bergamo, nello specifico presso lo Studio Tecnico Bettoni di Bettoni Massimo in Pisogne (Bs) [DOC 4]. Luglio – Settembre 2004 - Inizio collaborazione con lo Studio di Ingegneria del Dott.Ing. Giovan Battista Vigani con studio in Sovere (Bg), che si protrae fino al settembre 2004 [REF 4]. Aprile 2005 – Dicembre 2006 - Collaborazione con Studio Tecnico Arch. Ivano Alberto Mancini con studio in Schilpario (Bg). Settembre 2007 – Maggio 2010 - Impiegato tecnico presso Impresa Edile e Stradale Paccani S.p.A. con sede in Ardesio (Bg) [REF 5]. Ottobre 2008 - Tirocinio formativo presso Laboratorio di Prove sui Materiali del Dipartimento di Progettazione e Tecnologie dell’Università degli Studi di Bergamo. Dott. Ing. Lorenzo Ferrari Via Cornasola n.8/a – 24065 Lovere (Bergamo) Aprile 2010 – Dicembre 2010 - Collaborazione con Officina Meccanica con sede in Brusaporto (Bg) per la progettazione dei sistemi di supporto per impianti fotovoltaici e gestione dei cantieri. Ottobre 2013 (attuale collaborazione) - Istruttore tecnico presso l’ufficio Lavori Pubblici, manutenzioni, patrimonio, energia del Comune di Palazzolo sull’Oglio (Bs). - Diploma di maturità conseguito all’I.T.C.G. “T. Olivelli” di Darfo Istruzione Boario Terme (Bs) con votazione finale di 95/100. Lingua estera studiata: inglese [IST 3]. - Laurea triennale in Ingegneria Edile, conseguita presso l’Università degli Studi di Bergamo, Facoltà di Ingegneria, sede di Dalmine (Bg), con votazione finale di 103/110 [IST 4]. -
Franciacorta Shines with Chardonnay
PETER DRY Franciacorta shines with Chardonnay HERE IS Franciacorta? You might well ask. Outside of Italy the region and its wines are W virtually unknown. Although wine has been produced here since ancient times, the modern wine indus- try is young by Italian standards. Until the 1960s, the pleas- ant but undistinguished table wines of the region were con- sumed locally. Today, the region is synonymous with high quality sparkling wine. The development of the sparkling wine industry here is an interesting case study because it appears to have been one of those rare instances where the end product was chosen before the suitability of the grape material had been confirmed. For example, two major Italian wine companies, Bellavista and Ca’ del Bosco, decided to invest in the region (for economic reasons) in order to expand their production of sparkling wine. The market demand for the resultant product was good and, fortuitously, the region turned out to be a good location for Chardonnay grapes for sparkling wine. The vineyards of Franciacorta are found in rolling hills to the south of Lake Iseo in central Lombardy, 20 km Peter Dry west of Brescia and 60 km east of Milan. Lombardy is the Vineyards of the World largest and most populous region of Italy. In addition to Franciacorta, its wine-producing regions include Oltrepò Paese in the southwest, Valtellina in the north and Lugana and Riviera del Garda Bresciano in the east. The munities ( curtes ) were allowed to be free ( francae ) of taxes. name of the region may be derived from Francae curtes , a Since 1995, use of the Franciacorta name has been permit- reference to the small communities of Benedictine ted solely for Denominazione di Origine Controllata e Garantita monks who settled in the region in 1100 AD—the com- (DOCG) sparkling wines made by the methode champenoise . -
THE RIVER OGLIO GREENWAY Tour in 4 Stops to Be Covered in 4 Days Or to Be Split at Will in Tours of One Day Or 2-3 Days
THE RIVER OGLIO GREENWAY Tour in 4 stops to be covered in 4 days or to be split at will in tours of one day or 2-3 days. WHAT YOU’LL DISCOVER In these tours and stops you will cycle along a portion of the RIVER OGLIO GREENWAY: a route that will take you from the mountains of the upper Camonica Valley (2) along the shores of Lake Iseo (5) reaching the vineyards of Franciacorta (6). This greenway was awarded as the most beautiful cycle path in Italy at the Italian Green Road Awards 2019, it starts from the Tonale Pass at an altitude of 1883 and ends in the plain, in the province of Mantua, with an altitude difference of 1862mt, spread along its 280 km. The colors of the different areas will accompany you in the description of these stops. The tour can be cycled completely or can be split into 1- or 2-3-days tours. STOP N° 1 UPPER CAMONICA VALLEY You will set off from Tonale Pass riding along a dirt road, then reach Ponte di Legno, a nice high mountain town in Camonica Valley, where the two springs Frigidolfo and Narcanello merge and create the River Oglio. Tonale Pass, that marks the border between Lombardy and Trentino regions, is surrounded by the mountains where soldiers fought WW1 at the foot of Mount Adamello. We will cycle across part of Camonica Valley, stopping at a typical Italian trattoria if you wish. Then on to the land of the prehistoric Camuni, the first Italian Unesco World Heritage site: the rock engravings. -
The Romantic Choice
Lago d’Iseo The Romantic Choice ALTO LAGO D’ISEO BASSO SEBINO E FRANCIACORTA SEBINO Un piacevole itinerario Un susseguirsi di tornanti conduce medievale di Castro e, subito dopo, si lo straordinario ciclo di affreschi del automobilistico permette una a Riva di Solto: merita una sosta il giunge a Lovere. La cittadina, uno de Romanino. Proseguendo, una serie prima conoscenza del Lago piccolo centro “I Borghi più belli di gallerie conduce a Marone, la città d’Iseo. Il percorso da noi descritto ini- storico, uno dei d’Italia”, è ricca di dell’olio, da cui è possibile deviare zia ad Iseo, la cittadina che dà il nome più pittoreschi edifici storici me- verso Zone per ammirare lo spettaco- al lago, ma il giro, naturalmente, si può del lago. Uscen- dievali e palazzi lo delle Piramidi di Erosione oppure, iniziare da ognuna delle località co- do dal paese si Il giro di pregio, tra i appena prima dell’abitato, scendere stiere. Lasciato Iseo e proseguendo in apre il maestoso quali l’Accademia a destra verso la piccola frazione di direzione sud si incontrano Clusane, spettacolo Tadini, elegante Vello, conosciuta per la bella pista caratteristico borgo conosciuto per dell’orrido del pinacoteca. ciclopedonale a lago. la specialità gastronomica della tinca Bogn, una sug- del Lago Allontanandosi al forno e Paratico, dove la bella pas- gestiva insena- momentane- Proseguendo sulla strada costiera, si seggiata a lago merita senz’altro una tura dove rocce amente dalla incontra Sale Marasino, dal bellissi- sosta. Oltrepassato il ponte si entra verticali si tuffano d’Iseo riva, si arriva a mo e panoramico entroterra collinare in Sarnico, elegante e vivace borgo a strapiombo nel Costa Volpino: un e Sulzano, piccolo centro dal quale commerciale.