Astragalus Cusickii Delimited
Total Page:16
File Type:pdf, Size:1020Kb
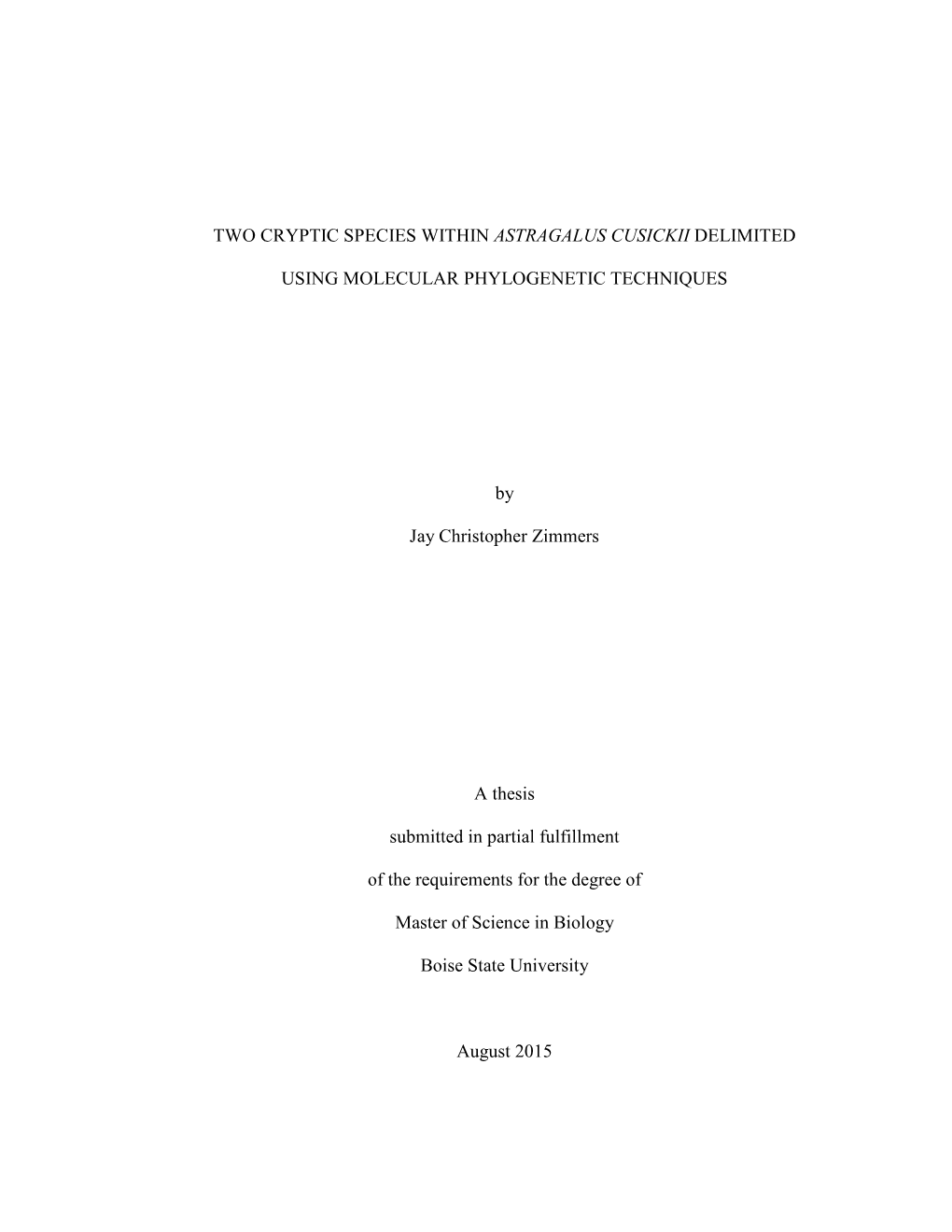
Load more
Recommended publications
-
Additional Information
Current Survey Introduced Flora Records Vegetation Condition *Acetosa vesicaria Excellent 534,000 mE 534,000 mE 535,000 534,000 mE 534,000 mE 535,000 534,000 mE 534,000 mE 535,000 534,000 mE 534,000 mE 535,000 534,000 mE 534,000 mE 535,000 534,000 mE 534,000 mE 535,000 534,000 mE 534,000 mE 535,000 536,000 mE 536,000 537,000 mE 537,000 536,000 mE 536,000 537,000 mE 537,000 536,000 mE 536,000 537,000 mE 537,000 536,000 mE 536,000 537,000 mE 537,000 536,000 mE 536,000 537,000 mE 537,000 536,000 mE 536,000 537,000 mE 537,000 536,000 mE 536,000 537,000 mE 537,000 534,000 mE 534,000 mE 535,000 534,000 mE 534,000 mE 535,000 534,000 mE 534,000 mE 535,000 534,000 mE 534,000 mE 535,000 534,000 mE 534,000 mE 535,000 534,000 mE 534,000 mE 535,000 534,000 mE 534,000 mE 535,000 536,000 mE 536,000 537,000 mE 537,000 536,000 mE 536,000 537,000 mE 537,000 536,000 mE 536,000 537,000 mE 537,000 536,000 mE 536,000 537,000 mE 537,000 536,000 mE 536,000 537,000 mE 537,000 536,000 mE 536,000 537,000 mE 537,000 536,000 mE 536,000 537,000 mE 537,000 534,000 mE 534,000 mE 535,000 534,000 mE 534,000 mE 535,000 534,000 mE 534,000 mE 535,000 534,000 mE 534,000 mE 535,000 534,000 mE 534,000 mE 535,000 534,000 mE 534,000 mE 535,000 534,000 mE 534,000 mE 535,000 536,000 mE 536,000 537,000 mE 537,000 536,000 mE 536,000 537,000 mE 537,000 536,000 mE 536,000 537,000 mE 537,000 536,000 mE 536,000 537,000 mE 537,000 536,000 mE 536,000 537,000 mE 537,000 536,000 mE 536,000 537,000 mE 537,000 536,000 mE 536,000 537,000 mE 537,000 534,000 mE 534,000 mE 535,000 534,000 mE 534,000 -
(Leguminosae): Nomenclatural Proposals and New Taxa
Great Basin Naturalist Volume 58 Number 1 Article 5 1-30-1998 Astragalus (Leguminosae): nomenclatural proposals and new taxa Stanley L. Welsh Brigham Young University Follow this and additional works at: https://scholarsarchive.byu.edu/gbn Recommended Citation Welsh, Stanley L. (1998) "Astragalus (Leguminosae): nomenclatural proposals and new taxa," Great Basin Naturalist: Vol. 58 : No. 1 , Article 5. Available at: https://scholarsarchive.byu.edu/gbn/vol58/iss1/5 This Article is brought to you for free and open access by the Western North American Naturalist Publications at BYU ScholarsArchive. It has been accepted for inclusion in Great Basin Naturalist by an authorized editor of BYU ScholarsArchive. For more information, please contact [email protected], [email protected]. Great Basin Naturalist 58(1), © 1998, pp. 45-53 ASTRAGALUS (LEGUMINOSAE): NOMENCLATURAL PROPOSALS AND NEW TAXA Stanley L. Welsh! ABSTRACT.-As part of an ongoing summary revision of Astragalus for the Flora North America project, several nomenclatural changes are indicated. Nomenclatural proposals include A. molybdenus val'. shultziorom (Barneby) Welsh, comb. nov.; A. australis var. aboriginorom (Richardson) Welsh, comb. nov.; A. australis var. cattoni (M.E. Jones) Welsh, comb. nov.; A. aU8tralis var. lepagei (Hulten) Welsh, comb. nov; A. australis var. muriei (Hulten) Welsh, comb. nov.; A. subcinereus var. sileranus (M.E. Jones) Welsh, comb. nov.; A. tegetariaides val'. anxius (Meinke & Kaye) Welsh, comb. nov.; A. ampullarioides (Welsh) Welsh, comb. nov.; A. cutlen (Barneby) Welsh, comb. nov.; and A. laccaliticus (M.E. Jones) Welsh, comb. nov. Proposals of new taxa include Astragalus sect. Scytocarpi subsect. Micl'ocymbi Welsh, subsed. nov., and A. sabulosus var. -
Plant Species of Special Concern and Vascular Plant Flora of the National
Plant Species of Special Concern and Vascular Plant Flora of the National Elk Refuge Prepared for the US Fish and Wildlife Service National Elk Refuge By Walter Fertig Wyoming Natural Diversity Database The Nature Conservancy 1604 Grand Avenue Laramie, WY 82070 February 28, 1998 Acknowledgements I would like to thank the following individuals for their assistance with this project: Jim Ozenberger, ecologist with the Jackson Ranger District of Bridger-Teton National Forest, for guiding me in his canoe on Flat Creek and for providing aerial photographs and lodging; Jennifer Whipple, Yellowstone National Park botanist, for field assistance and help with field identification of rare Carex species; Dr. David Cooper of Colorado State University, for sharing field information from his 1994 studies; Dr. Ron Hartman and Ernie Nelson of the Rocky Mountain Herbarium, for providing access to unmounted collections by Michele Potkin and others from the National Elk Refuge; Dr. Anton Reznicek of the University of Michigan, for confirming the identification of several problematic Carex specimens; Dr. Robert Dorn for confirming the identification of several vegetative Salix specimens; and lastly Bruce Smith and the staff of the National Elk Refuge for providing funding and logistical support and for allowing me free rein to roam the refuge for plants. 2 Table of Contents Page Introduction . 6 Study Area . 6 Methods . 8 Results . 10 Vascular Plant Flora of the National Elk Refuge . 10 Plant Species of Special Concern . 10 Species Summaries . 23 Aster borealis . 24 Astragalus terminalis . 26 Carex buxbaumii . 28 Carex parryana var. parryana . 30 Carex sartwellii . 32 Carex scirpoidea var. scirpiformis . -
Rail Development Vegetation and Flora Survey
JANUARY 2012 BROCKMAN RESOURCES LIMITED RAIL DEVELOPMENT VEGETATION AND FLORA SURVEY This page has been left blank intentionally BROCKMAN RESOURCES LIMITED RAIL DEVELOPMENT VEGETATION AND FLORA SURVEY Brockman Resources Limited Vegetation and Flora Survey Rail Corridor Document Status Approved for Issue Rev Author Reviewer Date Name Distributed To Date A Rochelle Renee 02/12/2011 Carol Macpherson Glenn Firth 02/12/2011 Haycock Tuckett Carol Macpherson B Carol 21.12.11 Carol Macpherson Glen Firth 23.12.11 Macpherson ecologia Environment (2011). Reproduction of this report in whole or in part by electronic, mechanical or chemical means including photocopying, recording or by any information storage and retrieval system, in any language, is strictly prohibited without the express approval of Brockman Resources Limited and/or ecologia Environment. Restrictions on Use This report has been prepared specifically for Brockman Resources Limited. Neither the report nor its contents may be referred to or quoted in any statement, study, report, application, prospectus, loan, or other agreement document, without the express approval of Brockman Resources Limited and/or ecologia Environment. ecologia Environment 1025 Wellington Street WEST PERTH WA 6005 Phone: 08 9322 1944 Fax: 08 9322 1599 Email: [email protected] December 2011 i Brockman Resources Limited Vegetation and Flora Survey Rail Corridor TABLE OF CONTENTS 1 INTRODUCTION ................................................................................................................ 1 -
Genetic Characterization of Three Varieties of Astragalus Lentiginosus (Fabaceae)
Genetic characterization of three varieties of Astragalus lentiginosus (Fabaceae) BRIANJ. KNAus,' RICHC. CRONN,AND AARONLISTON I Knaus, B. J. (Oregon State University, Department of Botany and Plant Pa- thology, 2082 Cordley Hall, Corvallis, OR, 97331-2902, U.S.A.; e-mail: [email protected]), R. C. Cronn (USDA Forest Service Pacific Northwest Research Station, 3200 SW Jefferson Way, Corvallis, OR, 97331, U.S .A.; e-mail: [email protected]) & A. Liston (Oregon State University, Depart- ment of Botany and Plant Pathology, 2082 Cordley Hall, Corvallis, OR, 97331- 2902, U.S.A.; e-mail: [email protected]).Genetic characterization of three varieties of Astragalus lentiginosus (Fabaceae). Brittonia 57: 334-344. 2005.-Astragalus lentiginosus is a polymorphic species that occurs in geologi- cally young habitats and whose varietal circumscription implies active morpho- logical and genetic differentiation. In this preliminary study, we evaluate the po- tential of amplified fragment length polymorphism (AFLP) markers to resolve infraspecific taxa in three varieties of Astragalus lentiginosus. Distance-based principle coordinate and neighbor-joining analyses result in clustering of individ- uals that is congruent with population origin and varietal circumscription. Anal- ysis of molecular variance of two Oregon varieties demonstrates that varietal categories account for 11% of the total variance; in contrast, geographic proximity does not contribute to the total variance. AFLPs demonstrate an ability to dis- criminate varieties of A. lentiginosus despite a potentially confounding geographic pattern, and may prove effective at inferring relationships throughout the group. Key words: AFLP, amplified fragment length polymorphism, Astragalus lenti- ginosus, genetic differentiation, infraspecific taxa. Astragalus lentiginosus Dougl. -
Iowa State Journal of Research 61.2
oufiiil of Research Volume 61, No. 2 ISSN0092-6345 November, 1986 ISJRA6 61(2) 153-296 1986 From the Editors . 153 ISELY, D. Leguminosae of the United States. Astragalus L.: IV. Species Summary N-Z.. 157 Book Reviews . 291 IOWA STATE JOURNAL OF RESEARCH Published under the auspices of the Vice President for Research, Iowa State University EDITOR .................................................. DUANE ISELY ASSOCIATE EDITOR .............................. KENNETH G. MADISON ASSOCIATE EDITOR ...................................... PAUL N. HINZ ASSOCIATE EDITOR . BRUCE W. MENZEL ASSOCIATE EDITOR ................................... RAND D. CONGER COMPOSITOR-ASSISTANT EDITOR ............... CHRISTINE V. McDANIEL Administrative Board N. L. Jacobson, Chairman J. E. Galejs, I. S. U. Library D. Isely, Editor W. H. Kelly, College of Sciences and Humanities W. R. Madden, Office of Business and Finance J. P. Mahlstede, Agriculture and Horne Economics Experiment Station W. M. Schmitt, Information Service G. K. Serovy, College of Engineering Editorial Board G. J. Musick, Associate Editor for Entomology, University of Arkansas Paul W. Unger, Associate Editor for Agronomy, USDA, Bushland, Texas Dwight W. Bensend, Associate Editor for Forestry, Hale, Missouri L. Glenn Smith, Associate Editor for Education, Northern Illinois Univ. Faye S. Yates, Promotion Specialist, I. S. U. Gerald Klonglan, Consultant for Sociology, I. S. U. All matters pertaining to subscriptions, remittances, etc. should be addressed to the Iowa State University Press, 2121 South State Avenue, Ames, Iowa 50010. Most back issues of the IOWA STATE JOURNAL OF RESEARCH are available. Single copies starting with Volume 55 are $7.50 each, plus postage. Prior issues are $4.50 each, plus postage. Because of limited stocks, payment is required prior to shipment. -
Vascular Plant and Vertebrate Inventory of Fort Bowie National Historic Site Vascular Plant and Vertebrate Inventory of Fort Bowie National Historic Site
Powell, Schmidt, Halvorson In Cooperation with the University of Arizona, School of Natural Resources Vascular Plant and Vertebrate Inventory of Fort Bowie National Historic Site Vascular Plant and Vertebrate Inventory of Fort Bowie National Historic Site Plant and Vertebrate Vascular U.S. Geological Survey Southwest Biological Science Center 2255 N. Gemini Drive Flagstaff, AZ 86001 Open-File Report 2005-1167 Southwest Biological Science Center Open-File Report 2005-1167 February 2007 U.S. Department of the Interior U.S. Geological Survey National Park Service In cooperation with the University of Arizona, School of Natural Resources Vascular Plant and Vertebrate Inventory of Fort Bowie National Historic Site By Brian F. Powell, Cecilia A. Schmidt , and William L. Halvorson Open-File Report 2005-1167 December 2006 USGS Southwest Biological Science Center Sonoran Desert Research Station University of Arizona U.S. Department of the Interior School of Natural Resources U.S. Geological Survey 125 Biological Sciences East National Park Service Tucson, Arizona 85721 U.S. Department of the Interior DIRK KEMPTHORNE, Secretary U.S. Geological Survey Mark Myers, Director U.S. Geological Survey, Reston, Virginia: 2006 For product and ordering information: World Wide Web: http://www.usgs.gov/pubprod Telephone: 1-888-ASK-USGS For more information on the USGS-the Federal source for science about the Earth, its natural and living resources, natural hazards, and the environment: World Wide Web:http://www.usgs.gov Telephone: 1-888-ASK-USGS Suggested Citation Powell, B. F, C. A. Schmidt, and W. L. Halvorson. 2006. Vascular Plant and Vertebrate Inventory of Fort Bowie National Historic Site. -
The Vascular Flora of the Upper Santa Ana River Watershed, San Bernardino Mountains, California
See discussions, stats, and author profiles for this publication at: https://www.researchgate.net/publication/281748553 THE VASCULAR FLORA OF THE UPPER SANTA ANA RIVER WATERSHED, SAN BERNARDINO MOUNTAINS, CALIFORNIA Article · January 2013 CITATIONS READS 0 28 6 authors, including: Naomi S. Fraga Thomas Stoughton Rancho Santa Ana B… Plymouth State Univ… 8 PUBLICATIONS 14 3 PUBLICATIONS 0 CITATIONS CITATIONS SEE PROFILE SEE PROFILE Available from: Thomas Stoughton Retrieved on: 24 November 2016 Crossosoma 37(1&2), 2011 9 THE VASCULAR FLORA OF THE UPPER SANTA ANA RIVER WATERSHED, SAN BERNARDINO MOUNTAINS, CALIFORNIA Naomi S. Fraga, LeRoy Gross, Duncan Bell, Orlando Mistretta, Justin Wood1, and Tommy Stoughton Rancho Santa Ana Botanic Garden 1500 North College Avenue Claremont, California 91711 1Aspen Environmental Group, 201 North First Avenue, Suite 102, Upland, California 91786 [email protected] All Photos by Naomi S. Fraga ABSTRACT: We present an annotated catalogue of the vascular flora of the upper Santa Ana River watershed, in the southern San Bernardino Mountains, in southern California. The catalogue is based on a floristic study, undertaken from 2008 to 2010. Approximately 65 team days were spent in the field and over 5,000 collections were made over the course of the study. The study area is ca. 155 km2 in area (40,000 ac) and ranges in elevation from 1402 m to 3033 m. The study area is botanically diverse with more than 750 taxa documented, including 56 taxa of conservation concern and 81 non-native taxa. Vegetation and habitat types in the area include chaparral, evergreen oak forest and woodland, riparian forest, coniferous forest, montane meadow, and pebble plain habitats. -
Anza-Borrego Desert State Park Bibliography Compiled and Edited by Jim Dice
Steele/Burnand Anza-Borrego Desert Research Center University of California, Irvine UCI – NATURE and UC Natural Reserve System California State Parks – Colorado Desert District Anza-Borrego Desert State Park & Anza-Borrego Foundation Anza-Borrego Desert State Park Bibliography Compiled and Edited by Jim Dice (revised 1/31/2019) A gaggle of geneticists in Borrego Palm Canyon – 1975. (L-R, Dr. Theodosius Dobzhansky, Dr. Steve Bryant, Dr. Richard Lewontin, Dr. Steve Jones, Dr. TimEDITOR’S Prout. Photo NOTE by Dr. John Moore, courtesy of Steve Jones) Editor’s Note The publications cited in this volume specifically mention and/or discuss Anza-Borrego Desert State Park, locations and/or features known to occur within the present-day boundaries of Anza-Borrego Desert State Park, biological, geological, paleontological or anthropological specimens collected from localities within the present-day boundaries of Anza-Borrego Desert State Park, or events that have occurred within those same boundaries. This compendium is not now, nor will it ever be complete (barring, of course, the end of the Earth or the Park). Many, many people have helped to corral the references contained herein (see below). Any errors of omission and comission are the fault of the editor – who would be grateful to have such errors and omissions pointed out! [[email protected]] ACKNOWLEDGEMENTS As mentioned above, many many people have contributed to building this database of knowledge about Anza-Borrego Desert State Park. A quantum leap was taken somewhere in 2016-17 when Kevin Browne introduced me to Google Scholar – and we were off to the races. Elaine Tulving deserves a special mention for her assistance in dealing with formatting issues, keeping printers working, filing hard copies, ignoring occasional foul language – occasionally falling prey to it herself, and occasionally livening things up with an exclamation of “oh come on now, you just made that word up!” Bob Theriault assisted in many ways and now has a lifetime job, if he wants it, entering these references into Zotero. -
Ventura County Plant Species of Local Concern
Checklist of Ventura County Rare Plants (Twenty-second Edition) CNPS, Rare Plant Program David L. Magney Checklist of Ventura County Rare Plants1 By David L. Magney California Native Plant Society, Rare Plant Program, Locally Rare Project Updated 4 January 2017 Ventura County is located in southern California, USA, along the east edge of the Pacific Ocean. The coastal portion occurs along the south and southwestern quarter of the County. Ventura County is bounded by Santa Barbara County on the west, Kern County on the north, Los Angeles County on the east, and the Pacific Ocean generally on the south (Figure 1, General Location Map of Ventura County). Ventura County extends north to 34.9014ºN latitude at the northwest corner of the County. The County extends westward at Rincon Creek to 119.47991ºW longitude, and eastward to 118.63233ºW longitude at the west end of the San Fernando Valley just north of Chatsworth Reservoir. The mainland portion of the County reaches southward to 34.04567ºN latitude between Solromar and Sequit Point west of Malibu. When including Anacapa and San Nicolas Islands, the southernmost extent of the County occurs at 33.21ºN latitude and the westernmost extent at 119.58ºW longitude, on the south side and west sides of San Nicolas Island, respectively. Ventura County occupies 480,996 hectares [ha] (1,188,562 acres [ac]) or 4,810 square kilometers [sq. km] (1,857 sq. miles [mi]), which includes Anacapa and San Nicolas Islands. The mainland portion of the county is 474,852 ha (1,173,380 ac), or 4,748 sq. -
Reproductive Ecology of Astragalus Filipes, a Great Basin
REPRODUCTIVE ECOLOGY OF ASTRAGALUS FILIPES, A GREAT BASIN RESTORATION LEGUME by Kristal M. Watrous A thesis submitted in partial fulfillment of the requirements for the degree of MASTER OF SCIENCE in Biology Approved: ________________________ _______________________ James H. Cane Edward W. Evans Major Professor Committee Member ________________________ _______________________ Eugene W. Schupp Byron R. Burnham Committee Member Dean of Graduate Studies UTAH STATE UNIVERSITY Logan, Utah 2010 ii ABSTRACT Reproductive Ecology of Astragalus filipes, a Great Basin Restoration Legume by Kristal M. Watrous, Master of Science Utah State University, 2010 Major Professor: Dr. James H. Cane Department: Biology Astragalus filipes Torrey ex. A. Gray (Fabaceae) is being studied and propagated for use in rangeland restoration projects throughout the Great Basin. Restoration forbs often require sufficient pollination services for seed production and persistence in restoration sites. Knowledge of a plant’s breeding biology is important in providing pollination for maximal seed set. Reproductive output from four manual pollination treatments (autogamy, geitonogamy, xenogamy, and distant xenogamy) was examined in a common garden. Pod set, seed set, and seed germination were quantified for each of the treatments. Seed set from four wild populations was compared to that of an openly visited common garden array. A. filipes was found to be self-compatible, but to benefit greatly from outcrossing. Less seed germinated from distantly outcrossed treatments than for any other treatment, indicating possible outbreeding depression. Common garden plants set less seed per pod than any wild population, possibly due to a depauperate pollinator guild in the common garden. iii Bees were surveyed at wild A. filipes populations to identify common pollinators. -
Mulford's Milkvetch (Astragalus Mulfordiae)
Mulford's milkvetch (Astragalus mulfordiae) ENDANGERED Flowers and fruit (left), habit (center), and habitat (right) of Mulford’s milkvetch. Photos by Andrea Thorpe (left) and Nancy Fredricks (center and right). If downloading images from this website, please credit the photographer. Family Fabaceae Plant description Mulford’s milkvetch is a perennial species with a long taproot and clustered, slender, wiry, thinly strigose stems, 3–20 cm long, arising from a woody, many-branched caudex. Leaves are 4–10 cm long including the petiole, with a flattened rachis and 11- 23 linear to elliptic leaflets, 0.3–0.8 cm long and nearly glabrous. Flowers are scattered, 5–20, in loose racemes on peduncles 5–8 cm long. The calyx is 0.28-0.5 cm long, strigose, with narrow triangular-subulate teeth slightly shorter than the tube. The corolla is whitish, drying yellow, the banner often bluish to purple lined or tinged, 0.5- 0.9 cm long. Pods are horizontally spreading, papery, inflated, 0.8-1.5 cm long, beaked, and almost triangular in cross-section. Valves are strigose and finely cross- veined, the ventral suture straight or curved slightly, the dorsal suture strongly curved. The slender stipe is 0.2-0.3 cm long. Distinguishing characteristics Mulford’s milkvetch is distinguished from the other Astragalus species with which it occurs by its small whitish flowers, its connate, sheath-forming lower stipules, and its pendulous, stipitate, three-faced pods. When to survey Surveys for Mulford’s milkvetch should be performed from May through June when the species is flowering and/or fruiting.