Combined Effects of Elevated Pco2 and Temperature on Biomass and Carbon
Total Page:16
File Type:pdf, Size:1020Kb
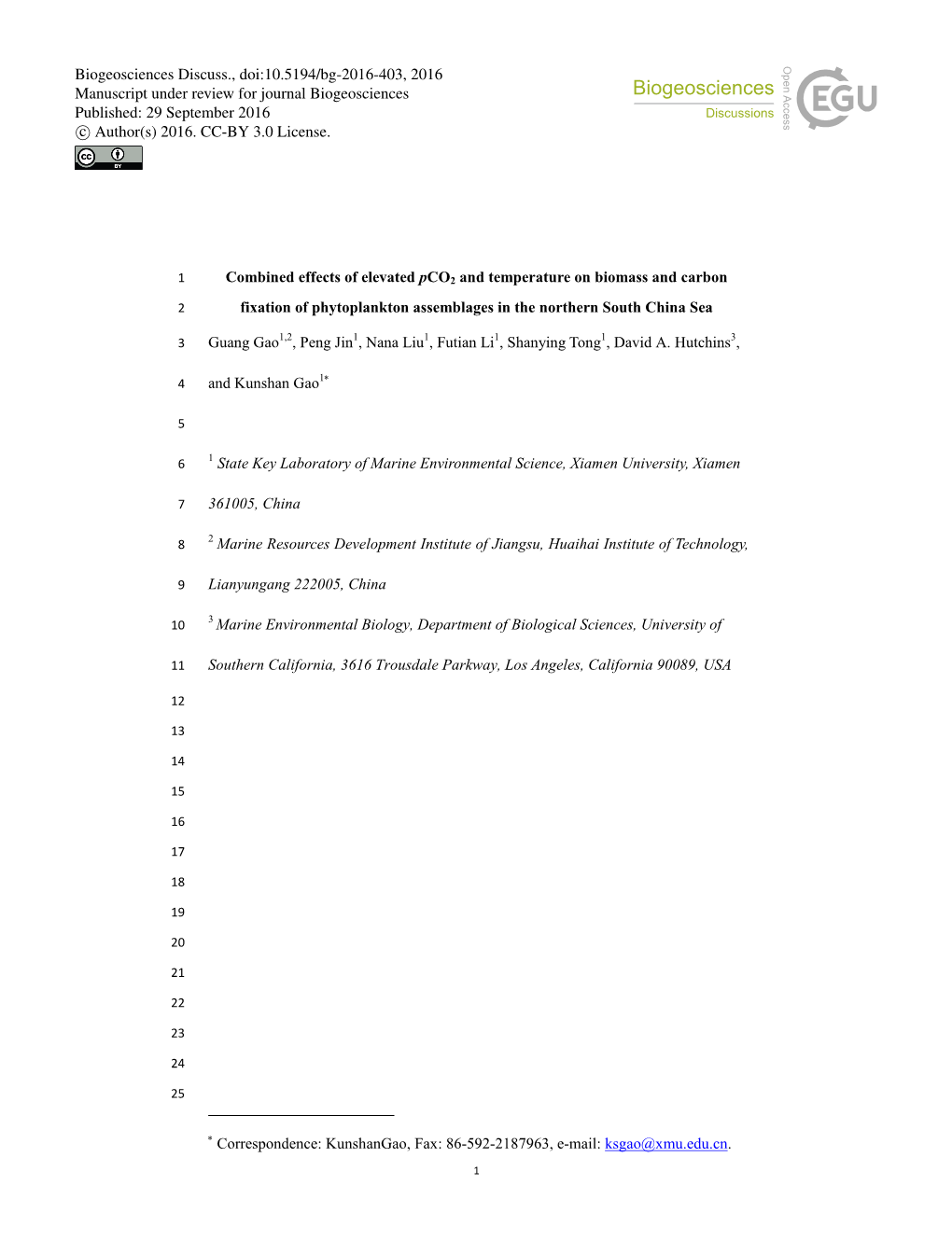
Load more
Recommended publications
-
7.014 Handout PRODUCTIVITY: the “METABOLISM” of ECOSYSTEMS
7.014 Handout PRODUCTIVITY: THE “METABOLISM” OF ECOSYSTEMS Ecologists use the term “productivity” to refer to the process through which an assemblage of organisms (e.g. a trophic level or ecosystem assimilates carbon. Primary producers (autotrophs) do this through photosynthesis; Secondary producers (heterotrophs) do it through the assimilation of the organic carbon in their food. Remember that all organic carbon in the food web is ultimately derived from primary production. DEFINITIONS Primary Productivity: Rate of conversion of CO2 to organic carbon (photosynthesis) per unit surface area of the earth, expressed either in terns of weight of carbon, or the equivalent calories e.g., g C m-2 year-1 Kcal m-2 year-1 Primary Production: Same as primary productivity, but usually expressed for a whole ecosystem e.g., tons year-1 for a lake, cornfield, forest, etc. NET vs. GROSS: For plants: Some of the organic carbon generated in plants through photosynthesis (using solar energy) is oxidized back to CO2 (releasing energy) through the respiration of the plants – RA. Gross Primary Production: (GPP) = Total amount of CO2 reduced to organic carbon by the plants per unit time Autotrophic Respiration: (RA) = Total amount of organic carbon that is respired (oxidized to CO2) by plants per unit time Net Primary Production (NPP) = GPP – RA The amount of organic carbon produced by plants that is not consumed by their own respiration. It is the increase in the plant biomass in the absence of herbivores. For an entire ecosystem: Some of the NPP of the plants is consumed (and respired) by herbivores and decomposers and oxidized back to CO2 (RH). -
Bacterial Production and Respiration
Organic matter production % 0 Dissolved Particulate 5 > Organic Organic Matter Matter Heterotrophic Bacterial Grazing Growth ~1-10% of net organic DOM does not matter What happens to the 90-99% of sink, but can be production is physically exported to organic matter production that does deep sea not get exported as particles? transported Export •Labile DOC turnover over time scales of hours to days. •Semi-labile DOC turnover on time scales of weeks to months. •Refractory DOC cycles over on time scales ranging from decadal to multi- decadal…perhaps longer •So what consumes labile and semi-labile DOC? How much carbon passes through the microbial loop? Phytoplankton Heterotrophic bacteria ?? Dissolved organic Herbivores ?? matter Higher trophic levels Protozoa (zooplankton, fish, etc.) ?? • Very difficult to directly measure the flux of carbon from primary producers into the microbial loop. – The microbial loop is mostly run on labile (recently produced organic matter) - - very low concentrations (nM) turning over rapidly against a high background pool (µM). – Unclear exactly which types of organic compounds support bacterial growth. Bacterial Production •Step 1: Determine how much carbon is consumed by bacteria for production of new biomass. •Bacterial production (BP) is the rate that bacterial biomass is created. It represents the amount of Heterotrophic material that is transformed from a nonliving pool bacteria (DOC) to a living pool (bacterial biomass). •Mathematically P = µB ?? µ = specific growth rate (time-1) B = bacterial biomass (mg C L-1) P= bacterial production (mg C L-1 d-1) Dissolved organic •Note that µ = P/B matter •Thus, P has units of mg C L-1 d-1 Bacterial production provides one measurement of carbon flow into the microbial loop How doe we measure bacterial production? Production (∆ biomass/time) (mg C L-1 d-1) • 3H-thymidine • 3H or 14C-leucine Note: these are NOT direct measures of biomass production (i.e. -
GLOBAL PRIMARY PRODUCTION and EVAPOTRANSPIRATION by Steven W
15 GLOBAL PRIMARY PRODUCTION AND EVAPOTRANSPIRATION by Steven W. Running and Maosheng Zhao DATA PRODUCTS Moderate Resolution Imaging Spectroradiometer Terrestrial primary production provides the energy to (MODIS) sensor, these variables are calculated maintain the structure and functions of ecosystems, every eight days in near real time at 1 km resolution. and supplies goods (e.g. food, fuel, wood and fi bre) MODIS GPP, NPP and ET data are available at the for human society. Gross primary production (GPP) EOS data gateway (see link below). is the amount of carbon fi xed by photosynthesis, To correct for contamination in the global and net primary production (NPP) is the amount of refl ectance data due to severe cloudiness or aerosols carbon converted into biomass after subtracting in the near real time products, these datasets are the cost of plant respiration. The water loss through reprocessed at the end of each year to build more exchange of trace gas CO2 by leaf stomata during stable, permanent datasets. These end-of-year photosynthesis plus evaporation from soil and versions of MODIS GPP, NPP and ET are available at plants is evapotranspiration (ET). ET computes the NTSG, University of Montana (see link below). water lost by a land surface, so it is consequently a component of the water balance in a region, and RESULTS FOR 2000–2006 is therefore relevant for drought monitoring and Figure 1 shows the seven-year average MODIS water management, providing an assessment of NPP for vegetated land on earth at 1 km spatial the water potentially available for human society. -
Phytoplankton As Key Mediators of the Biological Carbon Pump: Their Responses to a Changing Climate
sustainability Review Phytoplankton as Key Mediators of the Biological Carbon Pump: Their Responses to a Changing Climate Samarpita Basu * ID and Katherine R. M. Mackey Earth System Science, University of California Irvine, Irvine, CA 92697, USA; [email protected] * Correspondence: [email protected] Received: 7 January 2018; Accepted: 12 March 2018; Published: 19 March 2018 Abstract: The world’s oceans are a major sink for atmospheric carbon dioxide (CO2). The biological carbon pump plays a vital role in the net transfer of CO2 from the atmosphere to the oceans and then to the sediments, subsequently maintaining atmospheric CO2 at significantly lower levels than would be the case if it did not exist. The efficiency of the biological pump is a function of phytoplankton physiology and community structure, which are in turn governed by the physical and chemical conditions of the ocean. However, only a few studies have focused on the importance of phytoplankton community structure to the biological pump. Because global change is expected to influence carbon and nutrient availability, temperature and light (via stratification), an improved understanding of how phytoplankton community size structure will respond in the future is required to gain insight into the biological pump and the ability of the ocean to act as a long-term sink for atmospheric CO2. This review article aims to explore the potential impacts of predicted changes in global temperature and the carbonate system on phytoplankton cell size, species and elemental composition, so as to shed light on the ability of the biological pump to sequester carbon in the future ocean. -
Phytoplankton Primary Production and Its Utilization by the Pelagic Community in the Coastal Zone of the Gulf of Gdahsk (Southern Baltic)
MARINE ECOLOGY PROGRESS SERIES Vol. 148: 169-186. 1997 Published March 20 Mar Ecol Prog Ser l Phytoplankton primary production and its utilization by the pelagic community in the coastal zone of the Gulf of Gdahsk (southern Baltic) Zbigniew Witekl-*,Stanislaw Ochockil, Modesta ~aciejowska~, Marianna Pastuszakl, Jan ~akonieczny',Beata podgorska2, Janina M. ~ownacka', Tomasz Mackiewiczl, Magdalena Wrzesinska-Kwiecieh' 'Sea Fisheries Institute, ul. Koltqtaja 1, 81-332 Gdynia, Poland 'Marine Biology Center, Polish Academy of Sciences, ul. SW. Wojciecha S,81-347 Gdynia, Poland ABSTRACT In th~sstudy we estimated the amount and fate of phytoplankton primary product~onin the coastal zone of the Gulf of Gdansk, Poland, an area exposed to nutnent enrichment from the Vis- tuld R~verand nearby inunlcipal agglomeration The ~nvestigat~onswere carned out at 2 sites dur~ng 5 months in 1993 (February, April, May, August and October). A prolonged bloom pei-iod occurred in the coastal zone, as compared to the open Gulf and the open sea waters. From Aprll until October most values of gross primary production in the near-surface layer were in the range 100 to 500 mgC m-'' d1 Phytoplankton net exudate release constituted on average 5% of the gross prlmai-y production, total exudate release was estimated to be about 2 times h~gher.Bacterial production in the growth season was relatively low (the mean value ly~ngbetween 5 and 9%of gloss primary production), nevertheless, the microbial community (bactena and protozoans) ut~l~zeda large proportion of primary production (from about 50% in April and May to 16'%, in October). -
Sinking Jelly-Carbon Unveils Potential Environmental Variability Along a Continental Margin
Sinking Jelly-Carbon Unveils Potential Environmental Variability along a Continental Margin Mario Lebrato1,2*, Juan-Carlos Molinero1, Joan E. Cartes3, Domingo Lloris3, Fre´de´ric Me´lin4, Laia Beni- Casadella3 1 Department of Biogeochemistry and Ecology, Helmholtz Centre for Ocean Research Kiel (GEOMAR), Kiel, Germany, 2 Department of Geosciences, Scripps Institution of Oceanography, San Diego, California, United States of America, 3 Institut de Cie`ncies del Mar de Barcelona (CSIC), Barcelona, Spain, 4 Joint Research Centre, Ispra, Italy Abstract Particulate matter export fuels benthic ecosystems in continental margins and the deep sea, removing carbon from the upper ocean. Gelatinous zooplankton biomass provides a fast carbon vector that has been poorly studied. Observational data of a large-scale benthic trawling survey from 1994 to 2005 provided a unique opportunity to quantify jelly-carbon along an entire continental margin in the Mediterranean Sea and to assess potential links with biological and physical variables. Biomass depositions were sampled in shelves, slopes and canyons with peaks above 1000 carcasses per trawl, translating to standing stock values between 0.3 and 1.4 mg C m2 after trawling and integrating between 30,000 and 175,000 m2 of seabed. The benthopelagic jelly-carbon spatial distribution from the shelf to the canyons may be explained by atmospheric forcing related with NAO events and dense shelf water cascading, which are both known from the open Mediterranean. Over the decadal scale, we show that the jelly-carbon depositions temporal variability paralleled hydroclimate modifications, and that the enhanced jelly-carbon deposits are connected to a temperature-driven system where chlorophyll plays a minor role. -
Lecture 13 - Primary Production: Water Column Processes Prof
12.742 - Marine Chemistry Fall 2004 Lecture 13 - Primary Production: Water Column Processes Prof. Scott Doney Somewhat different organization from years past - start with surface productivity and work • our way down the water column Try to fold methods in as we go • Electronic notes still missing many of the figures - also somewhat different order. • Primary Production – Formation and respiration of organic matter Photosynthesis / respiration – Formation and dissolution of CaCO3, SiO3 Remineralization – Transport of dust, resuspended sediments, Dom Export particles – Scavenging onto particles Sinking Export – Elemental stoichiometry – Water column distributions – Rates Figure 1. hv Phototrophy – Categorize organisms by source of energy, inorganic electron donor and carbon source. CO + H O C + O 2 2 org 2 Chemo litho autotrophy · Chemo organo heterotrophy · Respiration Photo litho autotrophy Q (Chemical Energy) · Figure 2. 1 N N Light absorbed by pigments. • M – mainly chlorophyll a N N – also accessory pigments (which absorb different wavelengths) including forms of chlorophyll (b, c1, c2) carotenoids, biliopro- teins Figure 3. – Chlorophyll has conjugated double bonds - non-localized π orbital electrons, can absorb solar radiation, bump up to higher electron state – PAR - photosynthetic available radiation (350 700 nm) − – Convert light energy into electron energy 400 λ (nm) 700 – Light harvesting pigment-protein complexes Absorb in Blue and Red (leave Green) involved in electron transfer, funneling ex- cited electrons to reaction sites. -
Ocean Primary Production
Learning Ocean Science through Ocean Exploration Section 6 Ocean Primary Production Photosynthesis very ecosystem requires an input of energy. The Esource varies with the system. In the majority of ocean ecosystems the source of energy is sunlight that drives photosynthesis done by micro- (phytoplankton) or macro- (seaweeds) algae, green plants, or photosynthetic blue-green or purple bacteria. These organisms produce ecosystem food that supports the food chain, hence they are referred to as primary producers. The balanced equation for photosynthesis that is correct, but seldom used, is 6CO2 + 12H2O = C6H12O6 + 6H2O + 6O2. Water appears on both sides of the equation because the water molecule is split, and new water molecules are made in the process. When the correct equation for photosynthe- sis is used, it is easier to see the similarities with chemo- synthesis in which water is also a product. Systems Lacking There are some ecosystems that depend on primary Primary Producers production from other ecosystems. Many streams have few primary producers and are dependent on the leaves from surrounding forests as a source of food that supports the stream food chain. Snow fields in the high mountains and sand dunes in the desert depend on food blown in from areas that support primary production. The oceans below the photic zone are a vast space, largely dependent on food from photosynthetic primary producers living in the sunlit waters above. Food sinks to the bottom in the form of dead organisms and bacteria. It is as small as marine snow—tiny clumps of bacteria and decomposing microalgae—and as large as an occasional bonanza—a dead whale. -
Microbial Loop Carbon Cycling in Ocean Environments Studied Using a Simple Steady-State Model
AQUATIC MICROBIAL ECOLOGY Vol. 26: 37–49, 2001 Published October 26 Aquat Microb Ecol Microbial loop carbon cycling in ocean environments studied using a simple steady-state model Thomas R. Anderson1,*, Hugh W. Ducklow2 1Southampton Oceanography Centre, Waterfront Campus, European Way, Southampton SO14 3ZH, United Kingdom 2College of William and Mary School of Marine Science, Rte 1208, Box 1346, Gloucester Point, Virginia 23062, USA ABSTRACT: A simple steady-state model is used to examine the microbial loop as a pathway for organic C in marine systems, constrained by observed estimates of bacterial to primary production ratio (BP:PP) and bacterial growth efficiency (BGE). Carbon sources (primary production including extracellular release of dissolved organic carbon, DOC), cycling via zooplankton grazing and viral lysis, and sinks (bacterial and zooplankton respiration) are represented. Model solutions indicate that, at least under near steady-state conditions, recent estimates of BP:PP of about 0.1 to 0.15 are consistent with reasonable scenarios of C cycling (low BGE and phytoplankton extracellular release) at open ocean sites such as the Sargasso Sea and subarctic North Pacific. The finding that bacteria are a major (50%) sink for primary production is shown to be consistent with the best estimates of BGE and dissolved organic matter (DOM) production by zooplankton and phytoplankton. Zooplank- ton-related processes are predicted to provide the greatest supply of DOC for bacterial consumption. The bacterial contribution to C flow in the microbial loop, via bacterivory and viral lysis, is generally low, as a consequence of low BGE. Both BP and BGE are hard to quantify accurately. -
Deposition of Aerosols Onto Upper Ocean and Their Impacts on Marine Biota
atmosphere Review Deposition of Aerosols onto Upper Ocean and Their Impacts on Marine Biota Andreia Ventura 1,2, Eliana F. C. Simões 1 , Antoine S. Almeida 1 , Roberto Martins 2 , Armando C. Duarte 1 , Susana Loureiro 2 and Regina M. B. O. Duarte 1,* 1 CESAM—Centre for Environmental and Marine Studies, Department of Chemistry, University of Aveiro, 3810-193 Aveiro, Portugal; [email protected] (A.V.); [email protected] (E.F.C.S.); [email protected] (A.S.A.); [email protected] (A.C.D.) 2 CESAM—Centre for Environmental and Marine Studies, Department of Biology, University of Aveiro, 3810-193 Aveiro, Portugal; [email protected] (R.M.); [email protected] (S.L.) * Correspondence: [email protected] Abstract: Atmospheric aerosol deposition (wet and dry) is an important source of macro and micronu- trients (N, P, C, Si, and Fe) to the oceans. Most of the mass flux of air particles is made of fine mineral particles emitted from arid or semi-arid areas (e.g., deserts) and transported over long distances until deposition to the oceans. However, this atmospheric deposition is affected by anthropogenic activities, which heavily impacts the content and composition of aerosol constituents, contributing to the presence of potentially toxic elements (e.g., Cu). Under this scenario, the deposition of natural and anthropogenic aerosols will impact the biogeochemical cycles of nutrients and toxic elements in the ocean, also affecting (positively or negatively) primary productivity and, ultimately, the marine biota. Given the importance of atmospheric aerosol deposition to the oceans, this paper reviews the existing knowledge on the impacts of aerosol deposition on the biogeochemistry of the upper ocean, Citation: Ventura, A.; Simões, E.F.C.; and the different responses of marine biota to natural and anthropogenic aerosol input. -
Microbial Loop in an Oligotrophic Pelagic Marine Ecosystem: Possible Roles of Cyanobacteria and Nanoflagellates in the Organic Fluxes
MARINE ECOLOGY - PROGRESS SERIES Vol. 49: 171-178, 1988 Published November 10 Mar. Ecol. Prog. Ser. Microbial loop in an oligotrophic pelagic marine ecosystem: possible roles of cyanobacteria and nanoflagellates in the organic fluxes A. Hagstrom*, F. Azam**, A. Andersson*, J. Wikner*, F. Rassoulzadegan Station Marine de Villefranche-Sur-Mer, Station Zoologique. Universite P. et M. Curie, F-06230 Villefranche-Sur-Mer. France ABSTRACT: In an attempt to quantify the organic fluxes within the microbial loop of oligotrophic Mediterranean water, organic pools and production rates were monitored. The production of cyanobac- teria and its dynamics dominated the overall productivity in the system. The largest standing stock was that of the bacterioplankton and its growth consumed 8.3 pg C 1-' d-', hence about 60 % of the primary production was required for bacterial growth. Using the MiniCap technique, we measured a predation on bacteria of 2 6 X 104bacteria ml-' h-'. This was in good agreement with the bacterial production rate of 2.3 X 104 cells rnl-' h-' Thus, growth and predation were balanced for heterotrophic bacterioplank- ton. Almost all of this predation on bacteria was due to organisms passing a 12 vm Nuclepore filter. This raises the question of what mechanisms channel 60 % of primary production into bacteria. We therefore outlined a mass-balance model to illustrate routes that could explain this transfer. According to our model the main flux route is cyanobacteria and concomitantly consumed heterotrophic bacteria carbon into bacterivores. A substantial fraction of the bacterivore and the microplankton carbon is released by excretion and/or cell lysis, to be used by the heterotrophic bacterioplankton. -
Carbon Cycling and Biosequestration Workshop Report (Overview
1 • O Overview v e r v i e w DOE Workshop on Biological Carbon Cycling and Biosequestration Research he focus of climate research nationally and globally has shifted to estab lishing the capability to more accurately project climate change and its impacts, and to better define mitigation and adaptation options. The Tscience to achieve these new and much more challenging goals revolves around the development of Earth System Models (ESM) and the science to support them. These models combine physical climate models, global biological processes, and human activities. Understanding the global carbon cycle across terrestrial and ocean environments and its responses to climate change is essential for the viability of these models. The global carbon cycle is a balance between natural processes and emissions from human activities. This knowledge will provide the scientific under pinnings for more robust climate change modeling and help to identify carbon biosequestration–based mitigation strategies and human adaptation options over the coming decades. The Department of Energy’s (DOE) energy security mission is dependent on this modeling and research capability. Increasing atmospheric CO2 concentration is one of the most significant factors influ encing future climate. There has been a rapid accumulation of heat-trapping CO2 in the atmosphere [from 285 to 385 parts per million by volume (ppmv) since the Indus trial Revolution], largely due to human activities—primarily fossil energy use. Strate gies to minimize changes in climate will require that energy production and use be put in the context of Earth’s natural biogeochemical cycling of carbon and other elements. The DOE Office of Biological and Environmental Research (OBER) programs focus on increasing our understanding of carbon cycling in Earth’s marine and terrestrial ecosystems, examining potential means of biological sequestration of carbon, and determining how climate change affects biological processes that influ ence carbon cycling and biosequestration (altered carbon cycling in managed eco systems).