Random Splicing of Several Exons Caused by a Single Base Change in the Target Exon of CRISPR/Cas9 Mediated Gene Knockout
Total Page:16
File Type:pdf, Size:1020Kb
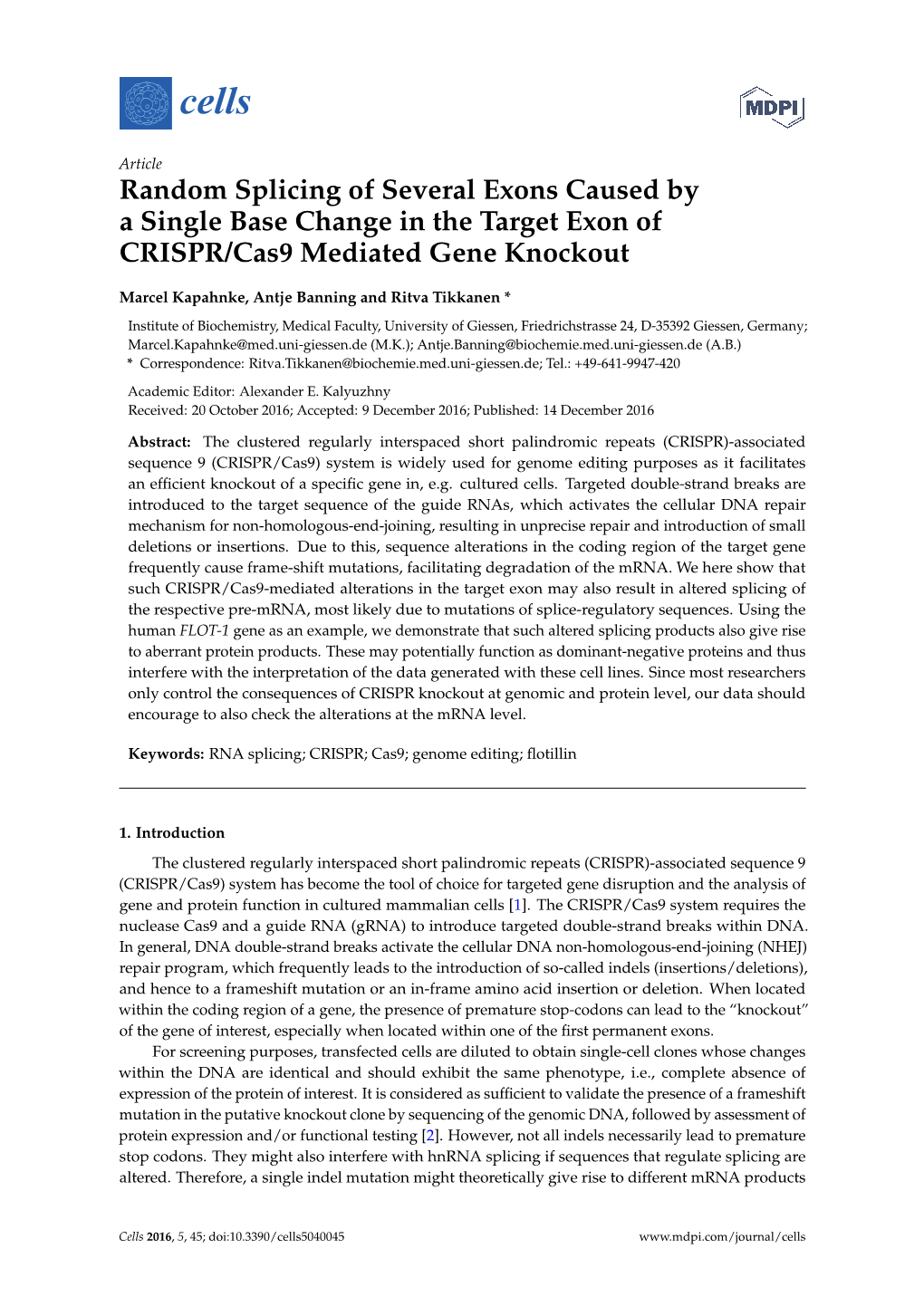
Load more
Recommended publications
-
Evolution, Expression and Meiotic Behavior of Genes Involved in Chromosome Segregation of Monotremes
G C A T T A C G G C A T genes Article Evolution, Expression and Meiotic Behavior of Genes Involved in Chromosome Segregation of Monotremes Filip Pajpach , Linda Shearwin-Whyatt and Frank Grützner * School of Biological Sciences, The University of Adelaide, Adelaide, SA 5005, Australia; fi[email protected] (F.P.); [email protected] (L.S.-W.) * Correspondence: [email protected] Abstract: Chromosome segregation at mitosis and meiosis is a highly dynamic and tightly regulated process that involves a large number of components. Due to the fundamental nature of chromosome segregation, many genes involved in this process are evolutionarily highly conserved, but duplica- tions and functional diversification has occurred in various lineages. In order to better understand the evolution of genes involved in chromosome segregation in mammals, we analyzed some of the key components in the basal mammalian lineage of egg-laying mammals. The chromosome passenger complex is a multiprotein complex central to chromosome segregation during both mitosis and meio- sis. It consists of survivin, borealin, inner centromere protein, and Aurora kinase B or C. We confirm the absence of Aurora kinase C in marsupials and show its absence in both platypus and echidna, which supports the current model of the evolution of Aurora kinases. High expression of AURKBC, an ancestor of AURKB and AURKC present in monotremes, suggests that this gene is performing all necessary meiotic functions in monotremes. Other genes of the chromosome passenger complex complex are present and conserved in monotremes, suggesting that their function has been preserved Citation: Pajpach, F.; in mammals. -
Detection Rate. FC: Fold Change. GO: Gene Ontology. AUC: Area Under
Figure S1. Flow chart of the study. DR: detection rate. FC: fold change. GO: Gene Ontology. AUC: area under curve. KEGG: Kyoto Encyclopedia of Genes and Genomes. GEO: Gene Expression Omnibus. Figure S2. Independent validation of miRNAs and the classifier. A, ΔCt of 6 selected miRNAs in the independent cohort. *, P < 0.05. **, P < 0.01. ***, P < 0.001. B, Receiver operating curve of the classifier in the independent cohort. AUC: area under curve. Figure S3. Venn of predicted target genes from 3 platforms. Figure S4. GO enrichment analysis. GO: Gene Ontology. Table S1. miRNA expression profile in screening stage. Table S2. miRNA expression profile in validation stage. Table S3. Efficacy of the classifier. Table S4. miRNA expression profile in independent validation stage. Table S5. Predicted target genes. Table S6a. KEGG enrichment analysis. KEGG: Kyoto Encyclopedia of Genes and Genomes. Table S6b. GO_BP enrichment analysis. GO: Gene Ontology. BP: Biological Process. Table S6c. GO_CC enrichment analysis. CC: Cellular Component. Table S6d. GO_MF enrichment analysis. MF: Molecular Function. Table S7. GEO expression array datasets involved in this study. GEO: Gene Expression Omnibus. Table S8a. Predicted target genes covered by the selected GEO datasets. GEO: Gene Expression Omnibus. 1 Table S8b. Expression profiles of predicted target genes of hsa-miR-26b-5p in GEO datasets. Table S8c. Expression profiles of predicted target genes of hsa-miR-146b-5p in GEO datasets. Table S8d. Expression profiles of predicted target genes of hsa-miR-191-5p in GEO datasets. Table S8e. Expression profiles of predicted target genes of hsa-miR-484 in GEO datasets. -
Flotillin 1 Antibody (R31142)
Flotillin 1 Antibody (R31142) Catalog No. Formulation Size R31142 0.5mg/ml if reconstituted with 0.2ml sterile DI water 100 ug Bulk quote request Availability 1-3 business days Species Reactivity Human, Mouse, Rat Format Antigen affinity purified Clonality Polyclonal (rabbit origin) Isotype Rabbit IgG Purity Antigen affinity Buffer Lyophilized from 1X PBS with 2.5% BSA and 0.025% sodium azide/thimerosal UniProt O75955 Applications Western blot : 0.5-1ug/ml Limitations This Flotillin 1 antibody is available for research use only. Western blot testing of Flotillin 1 antbody; Lane 1: rat lung; 2: (r) brain; 3: (r) ovary; 4: human SMMC-7721; 5: (h) MFC-7 cell lysate. Expected/observed molecular weight ~49kDa. Description Flotillin 1 is a protein that in humans is encoded by the FLOT1 gene. The International Radiation Hybrid Mapping Consortium mapped the gene to chromosome 6. Bickel et al.(1997) found that mouse Flot1 behaves as a resident integral membrane protein of caveolae. It consistently copurified with Flot2 and with caveolin-1 in the purification of caveolin-rich membranes. Hazarika et al.(1999) found that stable transfection of Flot1, which they called ESA/flotillin-2, in COS-1 cells induced filopodia formation and changed the epithelial morphology to that of neuronal cells. Santamaria et al.(2005) found that prostate tumor overexpressed gene-1 interacted with Flotillin1 in detergent-insoluble membrane fractions. Flotillin1 colocalized with PTOV1 at the plasma membrane and in the nucleus, and it entered the nucleus concomitant with PTOV1 shortly before initiation of S phase. Application Notes The stated application concentrations are suggested starting amounts. -
Snipa Snpcard
SNiPAcard Block annotations Block info genomic range chr6:29,970,960-30,792,117 block size 821,158 bp variant count 107 variants Basic features Conservation/deleteriousness Linked genes μ = -0.141 [-3.802 – ABCF1 , AL662797.1 , AL662800.2 , ATAT1 , C6orf136 , DHX16 , FLOT1 4.141] , GNL1 , HCG17 , HCG18 , HCG19P , HCG20 , HCG4P3 , HLA-J , HLA-L , HLA-N , IER3 , LINC00243 , MDC1 , MICC , MRPS18B , NRM , PAIP1P1 , PPP1R10 , PPP1R11 , PPP1R18 , PTMAP1 , RN7SL353P , phyloP gene(s) hit or close-by RNF39 , RPP21 , SUCLA2P1 , TRIM10 , TRIM15 , TRIM26 , TRIM26BP , TRIM31 , TRIM31-AS1 , TRIM39 , TRIM39-RPP21 , TRIM40 , TUBB , UBQLN1P1 , XXbac-BPG252P9.10 , XXbac-BPG252P9.9 , XXbac-BPG283O16.9 , Y_RNA , ZNRD1 , ZNRD1-AS1 , ZNRD1-AS1_3 μ = 0.130 [0 – 1] BTN3A2 , BTN3A3 , C2 , CCHCR1 , CFB , FLOT1 , GABBR1 , HCG17 , HCG20 , HCG22 , HCP5 , HIST1H3I , HLA-A , HLA-B , HLA-C , HLA-F- AS1 , HLA-G , HLA-H , HLA-J , HLA-K , HLA-L , HLA-U , HLA-V , IER3 phastCons eQTL gene(s) , LINC00243 , MICB , NDUFS1 , OR5V1 , PPP1R18 , PSORS1C1 , RNF39 , RPP21 , TMEM154 , TRIM26BP , TRIM31 , TRIM39-RPP21 , VARS2 , WASF5P , ZFP57 , ZNRD1 , ZNRD1-AS1_3 μ = -0.462 [-9.98 – 5.1] ATAT1 , ATAT1 , ATAT1 , ATAT1 , ATAT1 , ATAT1 , ATAT1 , DDR1 , DDR1 , DDR1 , DDR1 , DDR1 , DDR1 , DDR1 , FLOT1 , FLOT1 , FLOT1 , FLOT1 , FLOT1 , FLOT1 , FLOT1 , FLOT1 , HCG19P , HCG19P potentially regulated , HCG19P , HCG19P , HCG19P , HCG19P , HCG19P , NRM , NRM , GERP++ gene(s) NRM , NRM , NRM , NRM , NRM , RNF39 , RNF39 , RNF39 , RNF39 , RNF39 , RNF39 , RNF39 , RNF39 , TRIM26 , TRIM26 , TRIM26 -
Evolutionarily Conserved Intercalated Disc Protein Tmem65 Regulates Cardiac Conduction and Connexin 43 Function
ARTICLE Received 11 Aug 2014 | Accepted 18 Aug 2015 | Published 25 Sep 2015 DOI: 10.1038/ncomms9391 Evolutionarily conserved intercalated disc protein Tmem65 regulates cardiac conduction and connexin 43 function Parveen Sharma1,*, Cynthia Abbasi1,*, Savo Lazic2, Allen C.T. Teng1, Dingyan Wang1, Nicole Dubois3, Vladimir Ignatchenko4, Victoria Wong5, Jun Liu6, Toshiyuki Araki4, Malte Tiburcy7, Cameron Ackerley8, Wolfram H. Zimmermann7, Robert Hamilton8,11, Yu Sun6, Peter P. Liu9, Gordon Keller3, Igor Stagljar5, Ian C. Scott2,8,11, Thomas Kislinger4,10 & Anthony O. Gramolini1,11 Membrane proteins are crucial to heart function and development. Here we combine cationic silica-bead coating with shotgun proteomics to enrich for and identify plasma membrane-associated proteins from primary mouse neonatal and human fetal ventricular cardiomyocytes. We identify Tmem65 as a cardiac-enriched, intercalated disc protein that increases during development in both mouse and human hearts. Functional analysis of Tmem65 both in vitro using lentiviral shRNA-mediated knockdown in mouse cardiomyocytes and in vivo using morpholino-based knockdown in zebrafish show marked alterations in gap junction function and cardiac morphology. Molecular analyses suggest that Tmem65 interaction with connexin 43 (Cx43) is required for correct localization of Cx43 to the intercalated disc, since Tmem65 deletion results in marked internalization of Cx43, a shorter half-life through increased degradation, and loss of Cx43 function. Our data demonstrate that the membrane protein Tmem65 is an intercalated disc protein that interacts with and functionally regulates ventricular Cx43. 1 Department of Physiology, University of Toronto, Toronto General Hospital Research Institute, Toronto, Ontario, Canada M5G 1L7. 2 Department of Molecular Genetics, University of Toronto, Toronto, Ontario, Canada M5S 1A8. -
Fibroblasts from the Human Skin Dermo-Hypodermal Junction Are
cells Article Fibroblasts from the Human Skin Dermo-Hypodermal Junction are Distinct from Dermal Papillary and Reticular Fibroblasts and from Mesenchymal Stem Cells and Exhibit a Specific Molecular Profile Related to Extracellular Matrix Organization and Modeling Valérie Haydont 1,*, Véronique Neiveyans 1, Philippe Perez 1, Élodie Busson 2, 2 1, 3,4,5,6, , Jean-Jacques Lataillade , Daniel Asselineau y and Nicolas O. Fortunel y * 1 Advanced Research, L’Oréal Research and Innovation, 93600 Aulnay-sous-Bois, France; [email protected] (V.N.); [email protected] (P.P.); [email protected] (D.A.) 2 Department of Medical and Surgical Assistance to the Armed Forces, French Forces Biomedical Research Institute (IRBA), 91223 CEDEX Brétigny sur Orge, France; [email protected] (É.B.); [email protected] (J.-J.L.) 3 Laboratoire de Génomique et Radiobiologie de la Kératinopoïèse, Institut de Biologie François Jacob, CEA/DRF/IRCM, 91000 Evry, France 4 INSERM U967, 92260 Fontenay-aux-Roses, France 5 Université Paris-Diderot, 75013 Paris 7, France 6 Université Paris-Saclay, 78140 Paris 11, France * Correspondence: [email protected] (V.H.); [email protected] (N.O.F.); Tel.: +33-1-48-68-96-00 (V.H.); +33-1-60-87-34-92 or +33-1-60-87-34-98 (N.O.F.) These authors contributed equally to the work. y Received: 15 December 2019; Accepted: 24 January 2020; Published: 5 February 2020 Abstract: Human skin dermis contains fibroblast subpopulations in which characterization is crucial due to their roles in extracellular matrix (ECM) biology. -
Network Impact of the SERT Ala56 Coding Variant
fnmol-13-00089 June 8, 2020 Time: 16:4 # 1 ORIGINAL RESEARCH published: 08 June 2020 doi: 10.3389/fnmol.2020.00089 Ex vivo Quantitative Proteomic Analysis of Serotonin Transporter Interactome: Network Impact of the SERT Ala56 Coding Variant Meagan A. Quinlan1,2,3, Matthew J. Robson4, Ran Ye2, Kristie L. Rose5, Kevin L. Schey5 and Randy D. Blakely2,6* 1 Department of Psychiatry and Behavioral Sciences, University of Washington, Seattle, WA, United States, 2 Department of Pharmacology, Vanderbilt University, Nashville, TN, United States, 3 Department of Biomedical Science, Charles E. Schmidt College of Medicine, Florida Atlantic University, Jupiter, FL, United States, 4 Division of Pharmaceutical Sciences, James L. Winkle College of Pharmacy, University of Cincinnati, Cincinnati, OH, United States, 5 Department of Biochemistry, Vanderbilt University, Nashville, TN, United States, 6 Brain Institute, Florida Atlantic University, Jupiter, FL, United States Altered serotonin (5-HT) signaling is associated with multiple brain disorders, including major depressive disorder (MDD), obsessive-compulsive disorder (OCD), and autism spectrum disorder (ASD). The presynaptic, high-affinity 5-HT transporter (SERT) tightly regulates 5-HT clearance after release from serotonergic neurons in the brain and enteric nervous systems, among other sites. Accumulating evidence suggests that SERT is dynamically regulated in distinct activity states as a result of environmental and Edited by: Raul R. Gainetdinov, intracellular stimuli, with regulation perturbed by disease-associated coding variants. Saint Petersburg State University, Our lab identified a rare, hypermorphic SERT coding substitution, Gly56Ala, in Russia subjects with ASD, finding that the Ala56 variant stabilizes a high-affinity outward- Reviewed by: facing conformation (SERT∗) that leads to elevated 5-HT uptake in vitro and in vivo. -
Table S1 Genes with Coefficients of Variation (CV) Ratios (CVGHS/CVSD) in the Upper and Lower 10% of for Expression in Genetic Hypercalciuric Stone-Forming (GHS) And
Table S1 Genes with coefficients of variation (CV) ratios (CVGHS/CVSD) in the upper and lower 10% of for expression in Genetic Hypercalciuric Stone-forminG (GHS) and Sprague-Dawley (SD) rats, assayed usinG Affymetrix Rat Genome 230 GeneChips. The location of the microsatellite or quantitative trait locus (QTL) position (Loc) in base pairs (bp), gene initiation base pair (BP), chromosomal banding position and RGD or GenBank name are given.Rat Genome Database (RGD) (www.rgd.mcw.edu), GenBank (http://www.ncbi.nlm.nih.gov/Genbank/) and Entrez (http://www.ncbi.nlm.nih.gov/sites/entrez). Variance in gene expression was ranked according to CVGHS/CVSD ratio for all renal (8846 genes, Pctl, Renal) and duodenal genes (9038 genes, Pctl, Duod). Gene Pctl, Pctl, QTL Gene (GenBank) Renal Duod BP GenBank/RGD Description D1Mit95 Riok2 (BG371773) 91.3 52.4 55,897,246 RIO kinase 2 66,832,211 Tmc4 (BF545988) - 93.6 63,582,715 transmembrane channel-like gene family 4 Isoc2b (BM385414) 2.3 - 67,689,183 Isochorismatase domain containing 2b Suv420h2 (AW525235) 69.9 97.3 67,815,980 suppressor of variegation 4-20 homolog 2 (Drosophila) Lilrb4 (BF282961) 95.5 - 69,165,497 leukocyte immunoglobulin-like receptor, subfamily B, member 4 Zbtb45 (BI289556) - 10.0 72,912,203 zinc finger and BTB domain containing 45 Sepw1 (NM_013027) 7.5 - 76,249,869 selenoprotein W, muscle 1 Chmp2a (AW434104) 0.1 86.9 72,889,348 chromatin modifying protein 2a Tmem160 (AWS25031) 97.6 2.2 76,716,254 transmembrane protein Pnmal2 (BI282311) 99.6 - 77,277,341 PNMA-like 2 Dmpk (AI044427) 98.9 7.3 78,449,323 dystrophia myotonica-protein kinase; serine-threonine kinase Vasp (AW520792) 99.3 - 78,621,478 vasodilator-stimulated phosphoprotein Ercc1 (AA892791) 90.6 - 78,711,248 excision repair cross-complementing rodent repair deficiency, complementation G. -
List of Primer Oligos for Qpcr Analysis Gene Forward
SUPPLEMENTARY DATA Supplementary Table 1: List of Primer Oligos for qPCR analysis Gene Forward (5’->3’) Reverse (5’->3’) HPRT1 GAAAAGGACCCCACGAAGTGT AGTCAAGGGCATATCCTACAA B2M TGTCTTTCAGCAAGGACTGG AGCAAGCAAGCAGAATTTGG RPLP0 TGTTTCATTGTGGGAGCAGA GAACACAAAGCCCACATTCC GAPDH TGCACCACCAACTGCTTAGC GGCATGGACTGTGGTCATGAG DAPK3 AATCTGAGGAGCTGGGTTGC TCCAGGGAAAGTGCAGTCAC ATP2A3 AGTGCTCCGAAGACAACCC GTTCTCCGAGACGCTGTTG PPIB ATGTAGGCCGGGTGATCTTT CATCTCCCCTGGTGAAGTCT TBP AGTTCTGGGATTGTACCGCA TATATTCGGCGTTTCGGGCA mRNA was harvested from cultured myotubesusing a kit (E.Z.N.A. Total RNA Kit 1, Omega bio-tek, Norcross, GA). Reverse transcription and quantitative PCR were carried out using MultiScribe Reverse Transcriptase and Fast SYBR Green Master Mix, respectively (Thermo Fisher Scientific). Gene expression of ATP2A3, DAPK3, and reference genes (PPIB, TBP) was assessed using self-designed oligonucleotides (Sigma-Aldrich). ©2016 American Diabetes Association. Published online at http://diabetes.diabetesjournals.org/lookup/suppl/doi:10.2337/db16-0882/-/DC1 SUPPLEMENTARY DATA Supplementary Table 2: List of Antibodies used for Western-blot analysis Target Vendor Product Number PKB p-Thr-308 Cell Signaling (Danvers, MA) 4056 PKB total Cell Signaling 9272 TBC1D4 p-Ser-318 Cell Signaling 8619 TBC1D4 total Merck Millipore (Billerica, MA) 07-741 p62 Sigma-Aldrich P0067 LC3 Sigma-Aldrich L8918 GAPDH Santa Cruz Biotechnology (Dallas, TX) sc-257758 Myotube lysates were diluted in Laemmli buffer (60 mMtris base at pH 6.8, 2% w/v SDS, 10% v/v glycerol, 0.01% w/v bromophenol blue, 1.25% v/v β-mercaptoethanol). SDS-PAGE was conducted using pre-cast tris-HCl and tris-bis gels with tris/glycine/SDS or 2-(N-morpholino)ethanesulfonic acid running buffers, respectively (Bio-Rad). Proteins were transferred to Immobilon-P PVDF membranes (Merck Millipore). Equal loading of protein between samples was assessed by Ponceau staining (Sigma- Aldrich) or fluorescent-detection in the case of stain-free gels (Bio-Rad). -
Plant Flotillins Are Required for Infection by Nitrogen-Fixing Bacteria
Plant flotillins are required for infection by nitrogen-fixing bacteria Cara H. Haney and Sharon R. Long1 Department of Biology, Stanford University, Stanford, CA 94305-5020 Edited by Robert Haselkorn, University of Chicago, Chicago, IL, and approved November 12, 2009 (received for review September 2, 2009) To establish compatible rhizobial-legume symbioses, plant roots ponents of the proposed NF entry receptor pathway are currently support bacterial infection via host-derived infection threads (ITs). limited to NIN and LYK3; how LYK3 signaling is perceived and Here, we report the requirement of plant flotillin-like genes (FLOTs) translated into IT initiation is unknown. in Sinorhizobium meliloti infection of its host legume Medicago trun- Sinorhizobium meliloti, the nitrogen-fixing symbiont of Medi- catula. Flotillins in other organisms have roles in viral pathogenesis, cago (the alfalfa genus), is a close relative of the animal pathogen fi endocytosis, and membrane shaping. We identi ed seven FLOT Brucella. Sinorhizobium and Brucella have common genes re- genes in the M. truncatula genome and show that two, FLOT2 and quired for infection and invasion of their hosts (9). Given these FLOT4, are strongly up-regulated during early symbiotic events. This common features, we asked whether eukaryotic host-cell factors up-regulation depends on bacterial Nod Factor and the plant's ability required for infection might also be shared across kingdoms. to perceive Nod Factor. Microscopy data suggest that M. truncatula Upon uptake into host cells, Brucella remains surrounded by a FLOT2 and FLOT4 localize to membrane microdomains. Upon rhizo- fl bial inoculation, FLOT4 uniquely becomes localized to the tips of host-derived membrane, which contains the protein otillin (10). -
Analysis of Genomic Regions of Increased Gene Expression (RIDGE)S in Immune Activation
Analysis of genomic Regions of IncreaseD Gene Expression (RIDGE)s in immune activation Lena Hansson Doctor of Philosophy Institute for Adaptive and Neural Computation School of Informatics and Division of Pathway Medicine Medical School University of Edinburgh 2009 Abstract A RIDGE (Region of IncreaseD Gene Expression), as defined by previous studies, is a con- secutive set of active genes on a chromosome that span a region around 110 kbp long. This study investigated RIDGE formation by focusing on the well-defined, immunological impor- tant MHC locus. Macrophages were assayed for gene expression levels using the Affymetrix MG-U74Av2 chip are were either 1) uninfected, 2) primed with IFN-g, 3) viral activated with mCMV, or 4) both primed and viral activated. Gene expression data from these conditions was studied using data structures and new software developed for the visualisation and handling of structured functional genomic data. Specifically, the data was used to study RIDGE structures and investigate whether physically linked genes were also functionally related, and exhibited co-expression and potentially co-regulation. A greater number of RIDGEs with a greater number of members than expected by chance were found. Observed RIDGEs featured functional associations between RIDGE members (mainly explored via GO, UniProt, and Ingenuity), shared upstream control elements (via PROMO, TRANSFAC, and ClustalW), and similar gene expression profiles. Furthermore RIDGE formation cannot be explained by sequence duplication events alone. When the analysis was extended to the entire mouse genome, it became apparent that known genomic loci (for example the protocadherin loci) were more likely to contain more and longer RIDGEs. -
Membrane Partitioning by Flotillin-1 Facilitates Amphetamine-Induced Dopamine Transporter Activity
Membrane partitioning by Flotillin-1 facilitates amphetamine-induced dopamine transporter activity Wendy Mei Fong Submitted in partial fulfillment of the requirements for the degree of Doctor of Philosophy under the Executive Committee of the Graduate School of Arts and Sciences COLUMBIA UNIVERSITY 2017 © 2017 Wendy Mei Fong All rights reserved ABSTRACT Membrane partitioning by Flotillin-1 facilitates amphetamine-induced dopamine transporter activity Wendy Mei Fong Cellular membranes were once considered static and passive structures, but are now appreciated as a fluidic and dynamic assembly of macromolecules that play an active role in cellular function. Membrane composition has been proposed to play a critical role in modulating protein function by affecting everything from post-translational modifications to conformation, but the physiologic relevance of the relationship between protein and membrane has been difficult to establish. For example, membrane-associated proteins such as Flotillin-1 (Flot1) have been implicated to scaffold proteins into cholesterol-rich membranes, as well as play a role in a wide array of functions such as endocytosis and axon pathfinding; however, genetic elimination of Flot1 expression had little to no reported consequence, leaving to question the physiologic importance of scaffolding proteins to membrane microdomains. Using genetic and biochemical approaches, I sought to understand how the immediate lipid environment can influence the function of a transmembrane protein, and how this might impact brain function. Specifically, I examined how a cholesterol-rich environment can affect the function of the cell surface neurotransmitter transporter for dopamine, the dopamine transporter (DAT), and how this interaction may influence the ability of an organism to respond to the psychostimulant amphetamine (AMPH).