The Vacuum Impedance and Unit Systems
Total Page:16
File Type:pdf, Size:1020Kb
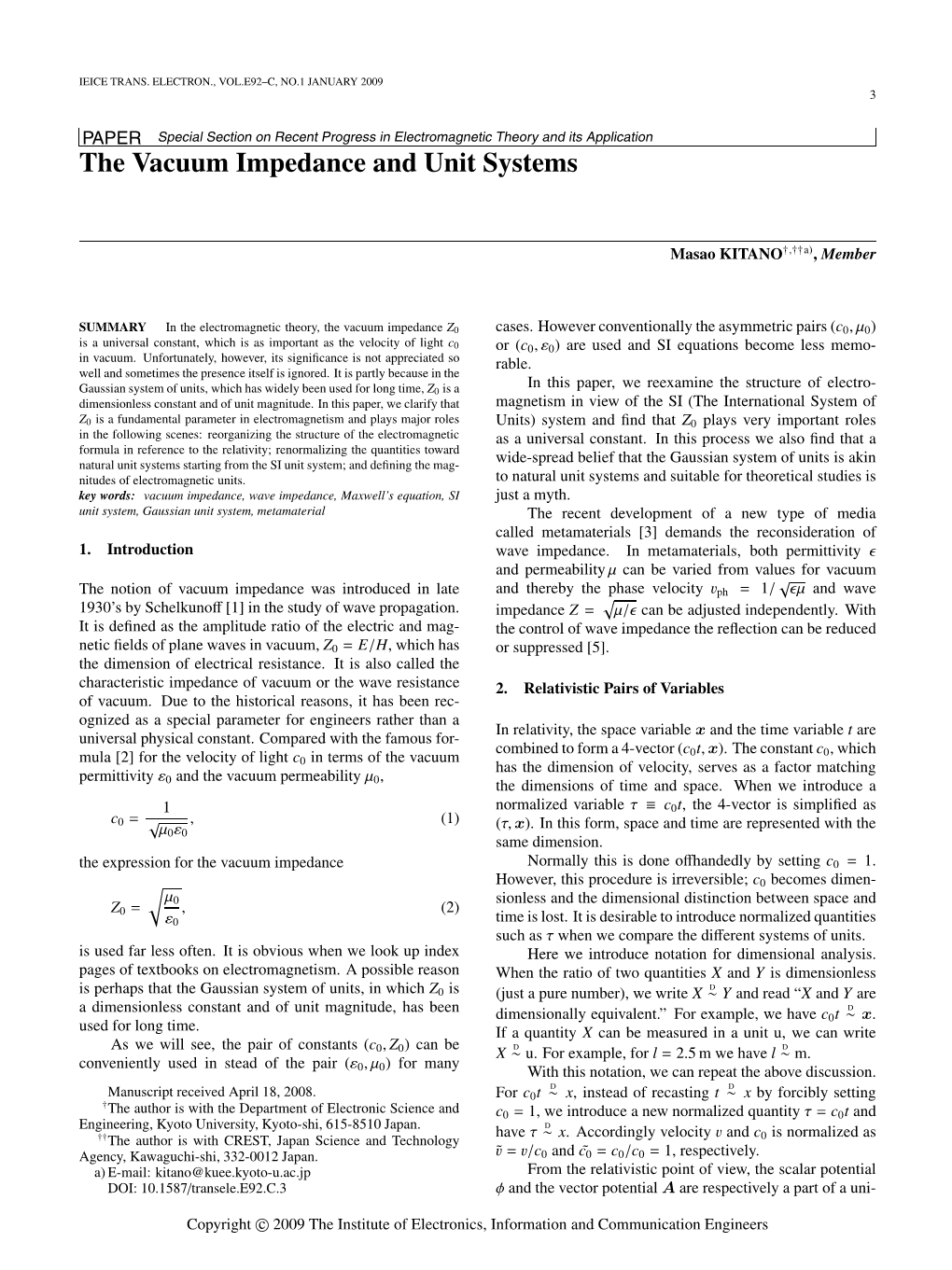
Load more
Recommended publications
-
Basic Magnetic Measurement Methods
Basic magnetic measurement methods Magnetic measurements in nanoelectronics 1. Vibrating sample magnetometry and related methods 2. Magnetooptical methods 3. Other methods Introduction Magnetization is a quantity of interest in many measurements involving spintronic materials ● Biot-Savart law (1820) (Jean-Baptiste Biot (1774-1862), Félix Savart (1791-1841)) Magnetic field (the proper name is magnetic flux density [1]*) of a current carrying piece of conductor is given by: μ 0 I dl̂ ×⃗r − − ⃗ 7 1 - vacuum permeability d B= μ 0=4 π10 Hm 4 π ∣⃗r∣3 ● The unit of the magnetic flux density, Tesla (1 T=1 Wb/m2), as a derive unit of Si must be based on some measurement (force, magnetic resonance) *the alternative name is magnetic induction Introduction Magnetization is a quantity of interest in many measurements involving spintronic materials ● Biot-Savart law (1820) (Jean-Baptiste Biot (1774-1862), Félix Savart (1791-1841)) Magnetic field (the proper name is magnetic flux density [1]*) of a current carrying piece of conductor is given by: μ 0 I dl̂ ×⃗r − − ⃗ 7 1 - vacuum permeability d B= μ 0=4 π10 Hm 4 π ∣⃗r∣3 ● The Physikalisch-Technische Bundesanstalt (German national metrology institute) maintains a unit Tesla in form of coils with coil constant k (ratio of the magnetic flux density to the coil current) determined based on NMR measurements graphics from: http://www.ptb.de/cms/fileadmin/internet/fachabteilungen/abteilung_2/2.5_halbleiterphysik_und_magnetismus/2.51/realization.pdf *the alternative name is magnetic induction Introduction It -
Admittance, Conductance, Reactance and Susceptance of New Natural Fabric Grewia Tilifolia V
Sensors & Transducers Volume 119, Issue 8, www.sensorsportal.com ISSN 1726-5479 August 2010 Editors-in-Chief: professor Sergey Y. Yurish, tel.: +34 696067716, fax: +34 93 4011989, e-mail: [email protected] Editors for Western Europe Editors for North America Meijer, Gerard C.M., Delft University of Technology, The Netherlands Datskos, Panos G., Oak Ridge National Laboratory, USA Ferrari, Vittorio, Universitá di Brescia, Italy Fabien, J. Josse, Marquette University, USA Katz, Evgeny, Clarkson University, USA Editor South America Costa-Felix, Rodrigo, Inmetro, Brazil Editor for Asia Ohyama, Shinji, Tokyo Institute of Technology, Japan Editor for Eastern Europe Editor for Asia-Pacific Sachenko, Anatoly, Ternopil State Economic University, Ukraine Mukhopadhyay, Subhas, Massey University, New Zealand Editorial Advisory Board Abdul Rahim, Ruzairi, Universiti Teknologi, Malaysia Djordjevich, Alexandar, City University of Hong Kong, Hong Kong Ahmad, Mohd Noor, Nothern University of Engineering, Malaysia Donato, Nicola, University of Messina, Italy Annamalai, Karthigeyan, National Institute of Advanced Industrial Science Donato, Patricio, Universidad de Mar del Plata, Argentina and Technology, Japan Dong, Feng, Tianjin University, China Arcega, Francisco, University of Zaragoza, Spain Drljaca, Predrag, Instersema Sensoric SA, Switzerland Arguel, Philippe, CNRS, France Dubey, Venketesh, Bournemouth University, UK Ahn, Jae-Pyoung, Korea Institute of Science and Technology, Korea Enderle, Stefan, Univ.of Ulm and KTB Mechatronics GmbH, Germany -
Series Impedance and Shunt Admittance Matrices of an Underground Cable System
SERIES IMPEDANCE AND SHUNT ADMITTANCE MATRICES OF AN UNDERGROUND CABLE SYSTEM by Navaratnam Srivallipuranandan B.E.(Hons.), University of Madras, India, 1983 A THESIS SUBMITTED IN PARTIAL FULFILLMENT OF THE REQUIREMENTS FOR THE DEGREE OF MASTER OF APPLIED SCIENCE in THE FACULTY OF GRADUATE STUDIES (Department of Electrical Engineering) We accept this thesis as conforming to the required standard THE UNIVERSITY OF BRITISH COLUMBIA, 1986 C Navaratnam Srivallipuranandan, 1986 November 1986 In presenting this thesis in partial fulfilment of the requirements for an advanced degree at the University of British Columbia, I agree that the Library shall make it freely available for reference and study. I further agree that permission for extensive copying of this thesis for scholarly purposes may be granted by the head of my department or by his or her representatives. It is understood that copying or publication of this thesis for financial gain shall not be allowed without my written permission. Department of The University of British Columbia 1956 Main Mall Vancouver, Canada V6T 1Y3 Date 6 n/8'i} SERIES IMPEDANCE AND SHUNT ADMITTANCE MATRICES OF AN UNDERGROUND CABLE ABSTRACT This thesis describes numerical methods for the: evaluation of the series impedance matrix and shunt admittance matrix of underground cable systems. In the series impedance matrix, the terms most difficult to compute are the internal impedances of tubular conductors and the earth return impedance. The various form u hit- for the interim!' impedance of tubular conductors and for th.: earth return impedance are, therefore, investigated in detail. Also, a more accurate way of evaluating the elements of the admittance matrix with frequency dependence of the complex permittivity is proposed. -
Magnetism Some Basics: a Magnet Is Associated with Magnetic Lines of Force, and a North Pole and a South Pole
Materials 100A, Class 15, Magnetic Properties I Ram Seshadri MRL 2031, x6129 [email protected]; http://www.mrl.ucsb.edu/∼seshadri/teach.html Magnetism Some basics: A magnet is associated with magnetic lines of force, and a north pole and a south pole. The lines of force come out of the north pole (the source) and are pulled in to the south pole (the sink). A current in a ring or coil also produces magnetic lines of force. N S The magnetic dipole (a north-south pair) is usually represented by an arrow. Magnetic fields act on these dipoles and tend to align them. The magnetic field strength H generated by N closely spaced turns in a coil of wire carrying a current I, for a coil length of l is given by: NI H = l The units of H are amp`eres per meter (Am−1) in SI units or oersted (Oe) in CGS. 1 Am−1 = 4π × 10−3 Oe. If a coil (or solenoid) encloses a vacuum, then the magnetic flux density B generated by a field strength H from the solenoid is given by B = µ0H −7 where µ0 is the vacuum permeability. In SI units, µ0 = 4π × 10 H/m. If the solenoid encloses a medium of permeability µ (instead of the vacuum), then the magnetic flux density is given by: B = µH and µ = µrµ0 µr is the relative permeability. Materials respond to a magnetic field by developing a magnetization M which is the number of magnetic dipoles per unit volume. The magnetization is obtained from: B = µ0H + µ0M The second term, µ0M is reflective of how certain materials can actually concentrate or repel the magnetic field lines. -
Impedance Matching
Impedance Matching Advanced Energy Industries, Inc. Introduction The plasma industry uses process power over a wide range of frequencies: from DC to several gigahertz. A variety of methods are used to couple the process power into the plasma load, that is, to transform the impedance of the plasma chamber to meet the requirements of the power supply. A plasma can be electrically represented as a diode, a resistor, Table of Contents and a capacitor in parallel, as shown in Figure 1. Transformers 3 Step Up or Step Down? 3 Forward Power, Reflected Power, Load Power 4 Impedance Matching Networks (Tuners) 4 Series Elements 5 Shunt Elements 5 Conversion Between Elements 5 Smith Charts 6 Using Smith Charts 11 Figure 1. Simplified electrical model of plasma ©2020 Advanced Energy Industries, Inc. IMPEDANCE MATCHING Although this is a very simple model, it represents the basic characteristics of a plasma. The diode effects arise from the fact that the electrons can move much faster than the ions (because the electrons are much lighter). The diode effects can cause a lot of harmonics (multiples of the input frequency) to be generated. These effects are dependent on the process and the chamber, and are of secondary concern when designing a matching network. Most AC generators are designed to operate into a 50 Ω load because that is the standard the industry has settled on for measuring and transferring high-frequency electrical power. The function of an impedance matching network, then, is to transform the resistive and capacitive characteristics of the plasma to 50 Ω, thus matching the load impedance to the AC generator’s impedance. -
Ec6503 - Transmission Lines and Waveguides Transmission Lines and Waveguides Unit I - Transmission Line Theory 1
EC6503 - TRANSMISSION LINES AND WAVEGUIDES TRANSMISSION LINES AND WAVEGUIDES UNIT I - TRANSMISSION LINE THEORY 1. Define – Characteristic Impedance [M/J–2006, N/D–2006] Characteristic impedance is defined as the impedance of a transmission line measured at the sending end. It is given by 푍 푍0 = √ ⁄푌 where Z = R + jωL is the series impedance Y = G + jωC is the shunt admittance 2. State the line parameters of a transmission line. The line parameters of a transmission line are resistance, inductance, capacitance and conductance. Resistance (R) is defined as the loop resistance per unit length of the transmission line. Its unit is ohms/km. Inductance (L) is defined as the loop inductance per unit length of the transmission line. Its unit is Henries/km. Capacitance (C) is defined as the shunt capacitance per unit length between the two transmission lines. Its unit is Farad/km. Conductance (G) is defined as the shunt conductance per unit length between the two transmission lines. Its unit is mhos/km. 3. What are the secondary constants of a line? The secondary constants of a line are 푍 i. Characteristic impedance, 푍0 = √ ⁄푌 ii. Propagation constant, γ = α + jβ 4. Why the line parameters are called distributed elements? The line parameters R, L, C and G are distributed over the entire length of the transmission line. Hence they are called distributed parameters. They are also called primary constants. The infinite line, wavelength, velocity, propagation & Distortion line, the telephone cable 5. What is an infinite line? [M/J–2012, A/M–2004] An infinite line is a line where length is infinite. -
A Collection of Definitions and Fundamentals for a Design-Oriented
A collection of definitions and fundamentals for a design-oriented inductor model 1st Andr´es Vazquez Sieber 2nd M´onica Romero * Departamento de Electronica´ * Departamento de Electronica´ Facultad de Ciencias Exactas, Ingenier´ıa y Agrimensura Facultad de Ciencias Exactas, Ingenier´ıa y Agrimensura Universidad Nacional de Rosario (UNR) Universidad Nacional de Rosario (UNR) ** Grupo Simulacion´ y Control de Sistemas F´ısicos ** Grupo Simulacion´ y Control de Sistemas F´ısicos CIFASIS-CONICET-UNR CIFASIS-CONICET-UNR Rosario, Argentina Rosario, Argentina [email protected] [email protected] Abstract—This paper defines and develops useful concepts related to the several kinds of inductances employed in any com- prehensive design-oriented ferrite-based inductor model, which is required to properly design and control high-frequency operated electronic power converters. It is also shown how to extract the necessary parameters from a ferrite material datasheet in order to get inductor models useful for a wide range of core temperatures and magnetic induction levels. Index Terms—magnetic circuit, ferrite core, major magnetic loop, minor magnetic loop, reversible inductance, amplitude inductance I. INTRODUCTION Errite-core based low-frequency-current biased inductors F are commonly found, for example, in the LC output filter of voltage source inverters (VSI) or step-down DC/DC con- verters. Those inductors have to effectively filter a relatively Fig. 1. General magnetic circuit low-amplitude high-frequency current being superimposed on a relatively large-amplitude low-frequency current. It is of practitioner. A design-oriented inductor model can be based paramount importance to design these inductors in a way that on the core magnetic model described in this paper which a minimum inductance value is always ensured which allows allows to employ the concepts of reversible inductance Lrevˆ , the accurate control and the safe operation of the electronic amplitude inductance La and initial inductance Li, to further power converter. -
Magnetic Properties of Materials Part 1. Introduction to Magnetism
Magnetic properties of materials JJLM, Trinity 2012 Magnetic properties of materials John JL Morton Part 1. Introduction to magnetism 1.1 Origins of magnetism The phenomenon of magnetism was most likely known by many ancient civil- isations, however the first recorded description is from the Greek Thales of Miletus (ca. 585 B.C.) who writes on the attraction of loadstone to iron. By the 12th century, magnetism is being harnessed for navigation in both Europe and China, and experimental treatises are written on the effect in the 13th century. Nevertheless, it is not until much later that adequate explanations for this phenomenon were put forward: in the 18th century, Hans Christian Ørsted made the key discovery that a compass was perturbed by a nearby electrical current. Only a week after hearing about Oersted's experiments, Andr´e-MarieAmp`ere, presented an in-depth description of the phenomenon, including a demonstration that two parallel wires carrying current attract or repel each other depending on the direction of current flow. The effect is now used to the define the unit of current, the amp or ampere, which in turn defines the unit of electric charge, the coulomb. 1.1.1 Amp`ere's Law Magnetism arises from charge in motion, whether at the microscopic level through the motion of electrons in atomic orbitals, or at macroscopic level by passing current through a wire. From the latter case, Amp`ere'sobservation was that the magnetising field H around any conceptual loop in space was equal to the current enclosed by the loop: I I = Hdl (1.1) By symmetry, the magnetising field must be constant if we take concentric circles around a current-carrying wire. -
Waveguides Waveguides, Like Transmission Lines, Are Structures Used to Guide Electromagnetic Waves from Point to Point. However
Waveguides Waveguides, like transmission lines, are structures used to guide electromagnetic waves from point to point. However, the fundamental characteristics of waveguide and transmission line waves (modes) are quite different. The differences in these modes result from the basic differences in geometry for a transmission line and a waveguide. Waveguides can be generally classified as either metal waveguides or dielectric waveguides. Metal waveguides normally take the form of an enclosed conducting metal pipe. The waves propagating inside the metal waveguide may be characterized by reflections from the conducting walls. The dielectric waveguide consists of dielectrics only and employs reflections from dielectric interfaces to propagate the electromagnetic wave along the waveguide. Metal Waveguides Dielectric Waveguides Comparison of Waveguide and Transmission Line Characteristics Transmission line Waveguide • Two or more conductors CMetal waveguides are typically separated by some insulating one enclosed conductor filled medium (two-wire, coaxial, with an insulating medium microstrip, etc.). (rectangular, circular) while a dielectric waveguide consists of multiple dielectrics. • Normal operating mode is the COperating modes are TE or TM TEM or quasi-TEM mode (can modes (cannot support a TEM support TE and TM modes but mode). these modes are typically undesirable). • No cutoff frequency for the TEM CMust operate the waveguide at a mode. Transmission lines can frequency above the respective transmit signals from DC up to TE or TM mode cutoff frequency high frequency. for that mode to propagate. • Significant signal attenuation at CLower signal attenuation at high high frequencies due to frequencies than transmission conductor and dielectric losses. lines. • Small cross-section transmission CMetal waveguides can transmit lines (like coaxial cables) can high power levels. -
Ee334lect37summaryelectroma
EE334 Electromagnetic Theory I Todd Kaiser Maxwell’s Equations: Maxwell’s equations were developed on experimental evidence and have been found to govern all classical electromagnetic phenomena. They can be written in differential or integral form. r r r Gauss'sLaw ∇ ⋅ D = ρ D ⋅ dS = ρ dv = Q ∫∫ enclosed SV r r r Nomagneticmonopoles ∇ ⋅ B = 0 ∫ B ⋅ dS = 0 S r r ∂B r r ∂ r r Faraday'sLaw ∇× E = − E ⋅ dl = − B ⋅ dS ∫∫S ∂t C ∂t r r r ∂D r r r r ∂ r r Modified Ampere'sLaw ∇× H = J + H ⋅ dl = J ⋅ dS + D ⋅ dS ∫ ∫∫SS ∂t C ∂t where: E = Electric Field Intensity (V/m) D = Electric Flux Density (C/m2) H = Magnetic Field Intensity (A/m) B = Magnetic Flux Density (T) J = Electric Current Density (A/m2) ρ = Electric Charge Density (C/m3) The Continuity Equation for current is consistent with Maxwell’s Equations and the conservation of charge. It can be used to derive Kirchhoff’s Current Law: r ∂ρ ∂ρ r ∇ ⋅ J + = 0 if = 0 ∇ ⋅ J = 0 implies KCL ∂t ∂t Constitutive Relationships: The field intensities and flux densities are related by using the constitutive equations. In general, the permittivity (ε) and the permeability (µ) are tensors (different values in different directions) and are functions of the material. In simple materials they are scalars. r r r r D = ε E ⇒ D = ε rε 0 E r r r r B = µ H ⇒ B = µ r µ0 H where: εr = Relative permittivity ε0 = Vacuum permittivity µr = Relative permeability µ0 = Vacuum permeability Boundary Conditions: At abrupt interfaces between different materials the following conditions hold: r r r r nˆ × (E1 − E2 )= 0 nˆ ⋅(D1 − D2 )= ρ S r r r r r nˆ × ()H1 − H 2 = J S nˆ ⋅ ()B1 − B2 = 0 where: n is the normal vector from region-2 to region-1 Js is the surface current density (A/m) 2 ρs is the surface charge density (C/m ) 1 Electrostatic Fields: When there are no time dependent fields, electric and magnetic fields can exist as independent fields. -
Wave Guides Summary and Problems
ECE 144 Electromagnetic Fields and Waves Bob York General Waveguide Theory Basic Equations (ωt γz) Consider wave propagation along the z-axis, with fields varying in time and distance according to e − . The propagation constant γ gives us much information about the character of the waves. We will assume that the fields propagating in a waveguide along the z-axis have no other variation with z,thatis,the transverse fields do not change shape (other than in magnitude and phase) as the wave propagates. Maxwell’s curl equations in a source-free region (ρ =0andJ = 0) can be combined to give the wave equations, or in terms of phasors, the Helmholtz equations: 2E + k2E =0 2H + k2H =0 ∇ ∇ where k = ω√µ. In rectangular or cylindrical coordinates, the vector Laplacian can be broken into two parts ∂2E 2E = 2E + ∇ ∇t ∂z2 γz so that with the assumed e− dependence we get the wave equations 2E +(γ2 + k2)E =0 2H +(γ2 + k2)H =0 ∇t ∇t (ωt γz) Substituting the e − into Maxwell’s curl equations separately gives (for rectangular coordinates) E = ωµH H = ωE ∇ × − ∇ × ∂Ez ∂Hz + γEy = ωµHx + γHy = ωEx ∂y − ∂y ∂Ez ∂Hz γEx = ωµHy γHx = ωEy − − ∂x − − − ∂x ∂Ey ∂Ex ∂Hy ∂Hx = ωµHz = ωEz ∂x − ∂y − ∂x − ∂y These can be rearranged to express all of the transverse fieldcomponentsintermsofEz and Hz,giving 1 ∂Ez ∂Hz 1 ∂Ez ∂Hz Ex = γ + ωµ Hx = ω γ −γ2 + k2 ∂x ∂y γ2 + k2 ∂y − ∂x w W w W 1 ∂Ez ∂Hz 1 ∂Ez ∂Hz Ey = γ + ωµ Hy = ω + γ γ2 + k2 − ∂y ∂x −γ2 + k2 ∂x ∂y w W w W For propagating waves, γ = β,whereβ is a real number provided there is no loss. -
Uniform Plane Waves
38 2. Uniform Plane Waves Because also ∂zEz = 0, it follows that Ez must be a constant, independent of z, t. Excluding static solutions, we may take this constant to be zero. Similarly, we have 2 = Hz 0. Thus, the fields have components only along the x, y directions: E(z, t) = xˆ Ex(z, t)+yˆ Ey(z, t) Uniform Plane Waves (transverse fields) (2.1.2) H(z, t) = xˆ Hx(z, t)+yˆ Hy(z, t) These fields must satisfy Faraday’s and Amp`ere’s laws in Eqs. (2.1.1). We rewrite these equations in a more convenient form by replacing and μ by: 1 η 1 μ = ,μ= , where c = √ ,η= (2.1.3) ηc c μ Thus, c, η are the speed of light and characteristic impedance of the propagation medium. Then, the first two of Eqs. (2.1.1) may be written in the equivalent forms: ∂E 1 ∂H ˆz × =− η 2.1 Uniform Plane Waves in Lossless Media ∂z c ∂t (2.1.4) ∂H 1 ∂E The simplest electromagnetic waves are uniform plane waves propagating along some η ˆz × = ∂z c ∂t fixed direction, say the z-direction, in a lossless medium {, μ}. The assumption of uniformity means that the fields have no dependence on the The first may be solved for ∂zE by crossing it with ˆz. Using the BAC-CAB rule, and transverse coordinates x, y and are functions only of z, t. Thus, we look for solutions noting that E has no z-component, we have: of Maxwell’s equations of the form: E(x, y, z, t)= E(z, t) and H(x, y, z, t)= H(z, t).