Developmental Regulation of Neuronal Gene Expression by Elongator Complex Protein 1
Total Page:16
File Type:pdf, Size:1020Kb
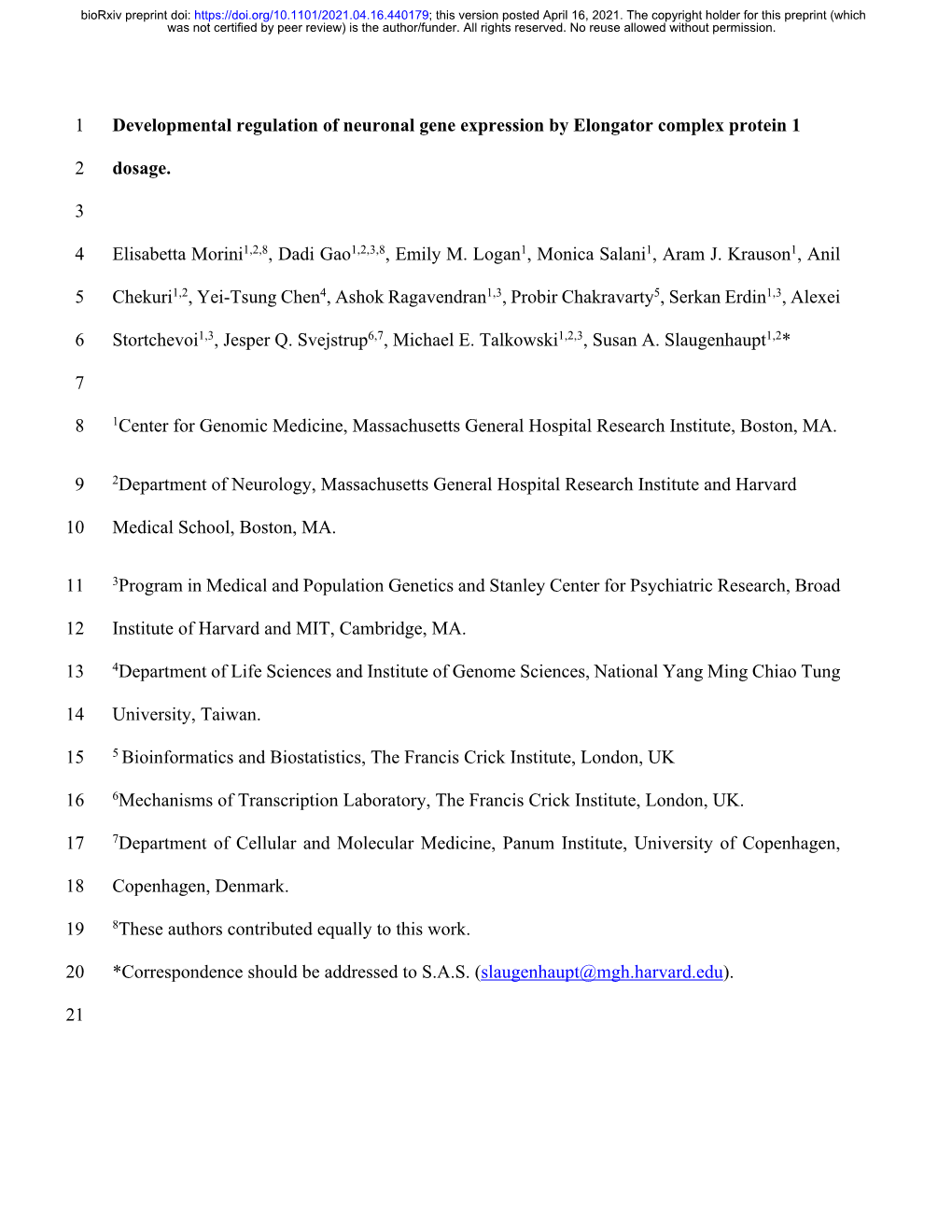
Load more
Recommended publications
-
In Human Metabolism
Supporting Information (SI Appendix) Framework and resource for more than 11,000 gene-transcript- protein-reaction associations (GeTPRA) in human metabolism SI Appendix Materials and Methods Standardization of Metabolite IDs with MNXM IDs Defined in the MNXref Namespace. Information on metabolic contents of the Recon 2Q was standardized using MNXM IDs defined in the MNXref namespace available at MetaNetX (1-3). This standardization was to facilitate the model refinement process described below. Each metabolite ID in the Recon 2Q was converted to MNXM ID accordingly. For metabolite IDs that were not converted to MNXM IDs, they were manually converted to MNXM IDs by comparing their compound structures and synonyms. In the final resulting SBML files, 97 metabolites were assigned with arbitrary IDs (i.e., “MNXMK_” followed by four digits) because they were not covered by the MNXref namespace (i.e., metabolite IDs not converted to MNXM IDs). Refinement or Removal of Biochemically Inconsistent Reactions. Recon 2 was built upon metabolic genes and reactions collected from EHMN (4, 5), the first genome-scale human liver metabolic model HepatoNet1 (6), an acylcarnitine and fatty-acid oxidation model Ac-FAO (7), and a small intestinal enterocyte model hs_eIEC611 (8). Flux variability analysis (9) of the Recon 2Q identified blocked reactions coming from these four sources of metabolic reaction data. The EHMN caused the greatest number of blocked reactions in the Recon 2Q (1,070 reactions corresponding to 69.3% of all the identified blocked reactions). To refine the EHMN reactions, following reactions were initially disregarded: 1) reactions having metabolite IDs not convertible to MNXM IDs; and 2) reactions without genes. -
Mitoxplorer, a Visual Data Mining Platform To
mitoXplorer, a visual data mining platform to systematically analyze and visualize mitochondrial expression dynamics and mutations Annie Yim, Prasanna Koti, Adrien Bonnard, Fabio Marchiano, Milena Dürrbaum, Cecilia Garcia-Perez, José Villaveces, Salma Gamal, Giovanni Cardone, Fabiana Perocchi, et al. To cite this version: Annie Yim, Prasanna Koti, Adrien Bonnard, Fabio Marchiano, Milena Dürrbaum, et al.. mitoXplorer, a visual data mining platform to systematically analyze and visualize mitochondrial expression dy- namics and mutations. Nucleic Acids Research, Oxford University Press, 2020, 10.1093/nar/gkz1128. hal-02394433 HAL Id: hal-02394433 https://hal-amu.archives-ouvertes.fr/hal-02394433 Submitted on 4 Dec 2019 HAL is a multi-disciplinary open access L’archive ouverte pluridisciplinaire HAL, est archive for the deposit and dissemination of sci- destinée au dépôt et à la diffusion de documents entific research documents, whether they are pub- scientifiques de niveau recherche, publiés ou non, lished or not. The documents may come from émanant des établissements d’enseignement et de teaching and research institutions in France or recherche français ou étrangers, des laboratoires abroad, or from public or private research centers. publics ou privés. Distributed under a Creative Commons Attribution| 4.0 International License Nucleic Acids Research, 2019 1 doi: 10.1093/nar/gkz1128 Downloaded from https://academic.oup.com/nar/advance-article-abstract/doi/10.1093/nar/gkz1128/5651332 by Bibliothèque de l'université la Méditerranée user on 04 December 2019 mitoXplorer, a visual data mining platform to systematically analyze and visualize mitochondrial expression dynamics and mutations Annie Yim1,†, Prasanna Koti1,†, Adrien Bonnard2, Fabio Marchiano3, Milena Durrbaum¨ 1, Cecilia Garcia-Perez4, Jose Villaveces1, Salma Gamal1, Giovanni Cardone1, Fabiana Perocchi4, Zuzana Storchova1,5 and Bianca H. -
Enhanced CRISPR-Based DNA Demethylation by Casilio-ME-Mediated RNA-Guided Coupling of Methylcytosine Oxidation and DNA Repair Pathways
ARTICLE https://doi.org/10.1038/s41467-019-12339-7 OPEN Enhanced CRISPR-based DNA demethylation by Casilio-ME-mediated RNA-guided coupling of methylcytosine oxidation and DNA repair pathways Aziz Taghbalout1, Menghan Du1, Nathaniel Jillette1, Wojciech Rosikiewicz1, Abhijit Rath2, Christopher D. Heinen2, Sheng Li1 & Albert W. Cheng 1,3,4* Casilio-ME 1234567890():,; Here we develop a methylation editing toolbox, , that enables not only RNA-guided methylcytosine editing by targeting TET1 to genomic sites, but also by co-delivering TET1 and protein factors that couple methylcytosine oxidation to DNA repair activities, and/or promote TET1 to achieve enhanced activation of methylation-silenced genes. Delivery of TET1 activity by Casilio-ME1 robustly alters the CpG methylation landscape of promoter regions and activates methylation-silenced genes. We augment Casilio-ME1 to simultaneously deliver the TET1-catalytic domain and GADD45A (Casilio-ME2) or NEIL2 (Casilio-ME3) to streamline removal of oxidized cytosine intermediates to enhance activation of targeted genes. Using two-in-one effectors or modular effectors, Casilio-ME2 and Casilio-ME3 remarkably boost gene activation and methylcytosine demethylation of targeted loci. We expand the toolbox to enable a stable and expression-inducible system for broader application of the Casilio-ME platforms. This work establishes a platform for editing DNA methylation to enable research investigations interrogating DNA methylomes. 1 The Jackson Laboratory for Genomic Medicine, 10 Discovery Drive, Farmington, CT 06032, USA. 2 Center for Molecular Oncology, University of Connecticut Health, 263 Farmington Avenue, Farmington, CT 06030, USA. 3 Department of Genetics and Genome Sciences, University of Connecticut Health, 400 Farmington Avenue, Farmington, CT 06030, USA. -
Supplementary Table 1: Genes Located on Chromosome 18P11-18Q23, an Area Significantly Linked to TMPRSS2-ERG Fusion
Supplementary Table 1: Genes located on Chromosome 18p11-18q23, an area significantly linked to TMPRSS2-ERG fusion Symbol Cytoband Description LOC260334 18p11 HSA18p11 beta-tubulin 4Q pseudogene IL9RP4 18p11.3 interleukin 9 receptor pseudogene 4 LOC100132166 18p11.32 hypothetical LOC100132166 similar to Rho-associated protein kinase 1 (Rho- associated, coiled-coil-containing protein kinase 1) (p160 LOC727758 18p11.32 ROCK-1) (p160ROCK) (NY-REN-35 antigen) ubiquitin specific peptidase 14 (tRNA-guanine USP14 18p11.32 transglycosylase) THOC1 18p11.32 THO complex 1 COLEC12 18pter-p11.3 collectin sub-family member 12 CETN1 18p11.32 centrin, EF-hand protein, 1 CLUL1 18p11.32 clusterin-like 1 (retinal) C18orf56 18p11.32 chromosome 18 open reading frame 56 TYMS 18p11.32 thymidylate synthetase ENOSF1 18p11.32 enolase superfamily member 1 YES1 18p11.31-p11.21 v-yes-1 Yamaguchi sarcoma viral oncogene homolog 1 LOC645053 18p11.32 similar to BolA-like protein 2 isoform a similar to 26S proteasome non-ATPase regulatory LOC441806 18p11.32 subunit 8 (26S proteasome regulatory subunit S14) (p31) ADCYAP1 18p11 adenylate cyclase activating polypeptide 1 (pituitary) LOC100130247 18p11.32 similar to cytochrome c oxidase subunit VIc LOC100129774 18p11.32 hypothetical LOC100129774 LOC100128360 18p11.32 hypothetical LOC100128360 METTL4 18p11.32 methyltransferase like 4 LOC100128926 18p11.32 hypothetical LOC100128926 NDC80 homolog, kinetochore complex component (S. NDC80 18p11.32 cerevisiae) LOC100130608 18p11.32 hypothetical LOC100130608 structural maintenance -
393LN V 393P 344SQ V 393P Probe Set Entrez Gene
393LN v 393P 344SQ v 393P Entrez fold fold probe set Gene Gene Symbol Gene cluster Gene Title p-value change p-value change chemokine (C-C motif) ligand 21b /// chemokine (C-C motif) ligand 21a /// chemokine (C-C motif) ligand 21c 1419426_s_at 18829 /// Ccl21b /// Ccl2 1 - up 393 LN only (leucine) 0.0047 9.199837 0.45212 6.847887 nuclear factor of activated T-cells, cytoplasmic, calcineurin- 1447085_s_at 18018 Nfatc1 1 - up 393 LN only dependent 1 0.009048 12.065 0.13718 4.81 RIKEN cDNA 1453647_at 78668 9530059J11Rik1 - up 393 LN only 9530059J11 gene 0.002208 5.482897 0.27642 3.45171 transient receptor potential cation channel, subfamily 1457164_at 277328 Trpa1 1 - up 393 LN only A, member 1 0.000111 9.180344 0.01771 3.048114 regulating synaptic membrane 1422809_at 116838 Rims2 1 - up 393 LN only exocytosis 2 0.001891 8.560424 0.13159 2.980501 glial cell line derived neurotrophic factor family receptor alpha 1433716_x_at 14586 Gfra2 1 - up 393 LN only 2 0.006868 30.88736 0.01066 2.811211 1446936_at --- --- 1 - up 393 LN only --- 0.007695 6.373955 0.11733 2.480287 zinc finger protein 1438742_at 320683 Zfp629 1 - up 393 LN only 629 0.002644 5.231855 0.38124 2.377016 phospholipase A2, 1426019_at 18786 Plaa 1 - up 393 LN only activating protein 0.008657 6.2364 0.12336 2.262117 1445314_at 14009 Etv1 1 - up 393 LN only ets variant gene 1 0.007224 3.643646 0.36434 2.01989 ciliary rootlet coiled- 1427338_at 230872 Crocc 1 - up 393 LN only coil, rootletin 0.002482 7.783242 0.49977 1.794171 expressed sequence 1436585_at 99463 BB182297 1 - up 393 -
The Role of H3K36 Methylation and Associated Methyltransferases In
bioRxiv preprint doi: https://doi.org/10.1101/2021.03.04.433843; this version posted March 5, 2021. The copyright holder for this preprint (which was not certified by peer review) is the author/funder. All rights reserved. No reuse allowed without permission. 1 The role of H3K36 methylation and associated 2 methyltransferases in chromosome‐specific gene regulation 3 4 Henrik Lindehell1, Alexander Glotov1, Eshagh Dorafshan1, Yuri B. Schwartz1 and Jan Larsson1* 5 1Department of Molecular Biology, Umeå University, SE‐90187 Umeå, Sweden 6 7 *Corresponding author: Jan Larsson, Department of Molecular Biology, Umeå University, SE‐ 8 90187 Umeå, Sweden, Tel: +46 (0)90 7856785; Fax: +46 (0)90 778007; Email: 9 [email protected] 10 11 Data deposition footnote: The RNA‐seq data reported in this paper have been deposited in 12 the Gene Expression Omnibus database (GSE166934). 1 bioRxiv preprint doi: https://doi.org/10.1101/2021.03.04.433843; this version posted March 5, 2021. The copyright holder for this preprint (which was not certified by peer review) is the author/funder. All rights reserved. No reuse allowed without permission. 13 Abstract 14 In Drosophila, two chromosomes require special mechanisms to balance their transcriptional 15 output to the rest of the genome. These are the male‐specific lethal complex targeting the 16 male X‐chromosome, and Painting of fourth targeting chromosome 4. The two systems are 17 evolutionarily linked to dosage compensation of the X‐chromosome and the chromosomes 18 involved display specific chromatin structures. Here we explore the role of histone H3 tri‐ 19 methylated at lysine 36 (H3K36me3) and the associated methyltransferases in these two 20 chromosome‐specific systems. -
The Role of NADP +
Order Number 9411942 The role of NADP+-malic enzyme in tomato fruit Finger, Fernando Luiz, Ph.D. The Ohio State University, 1993 Copyright ©1993 by Finger, Fernando Luiz. All rights reserved. U M I 300 N. Zeeb Rd. Ann Arbor, M I 48106 THE ROLE OF NADP+-MALIC ENZYME IN TOMATO FRUIT DISSERTATION Presented in Partial Fulfillment of the Requirements for the Degree Doctor of Philosophy in the Graduate School of The Ohio State University By Fernando Lufz Finger, B.S., M.S. ***** The Ohio State University 1993 Dissertation Committee: Approved by M. Knee L. M. Lagrimini [HLi t L ____ Advisor M. S. Biggs Department of Horticulture J. J. Finer Copyright by Fernando Lufz Finger 1993 To My Parents ii ACKNOWLEDGMENTS I would like to express my sincere appreciation to my advisor Dr. Michael Knee for his guidance and interest in this research. I would also like to thank Dr. Mark Lagrimini for sharing his expertise in molecular biology techniques. Thanks go to Drs. Scott Biggs and John Finer for their comments and suggestions. I would like to thank the following Brazilian institutions, Universidade Federal de Vigosa, CAPES and FAPEMIG for their financial support. Special thanks go to my colleague Dr. Raimundo Santos Barros for his friendship and encouragement. I am very grateful to Dr. Avtar Handa (Purdue University) for providing the tomato fruit lambda ZAP If library and Dr. Timothy Nelson (Yale University) for the corn malic enzyme cDNA clone. I would like to thank my fellow graduate students, in special, Joe Curran and Karen Klotz for their friendship. -
Table S1. 103 Ferroptosis-Related Genes Retrieved from the Genecards
Table S1. 103 ferroptosis-related genes retrieved from the GeneCards. Gene Symbol Description Category GPX4 Glutathione Peroxidase 4 Protein Coding AIFM2 Apoptosis Inducing Factor Mitochondria Associated 2 Protein Coding TP53 Tumor Protein P53 Protein Coding ACSL4 Acyl-CoA Synthetase Long Chain Family Member 4 Protein Coding SLC7A11 Solute Carrier Family 7 Member 11 Protein Coding VDAC2 Voltage Dependent Anion Channel 2 Protein Coding VDAC3 Voltage Dependent Anion Channel 3 Protein Coding ATG5 Autophagy Related 5 Protein Coding ATG7 Autophagy Related 7 Protein Coding NCOA4 Nuclear Receptor Coactivator 4 Protein Coding HMOX1 Heme Oxygenase 1 Protein Coding SLC3A2 Solute Carrier Family 3 Member 2 Protein Coding ALOX15 Arachidonate 15-Lipoxygenase Protein Coding BECN1 Beclin 1 Protein Coding PRKAA1 Protein Kinase AMP-Activated Catalytic Subunit Alpha 1 Protein Coding SAT1 Spermidine/Spermine N1-Acetyltransferase 1 Protein Coding NF2 Neurofibromin 2 Protein Coding YAP1 Yes1 Associated Transcriptional Regulator Protein Coding FTH1 Ferritin Heavy Chain 1 Protein Coding TF Transferrin Protein Coding TFRC Transferrin Receptor Protein Coding FTL Ferritin Light Chain Protein Coding CYBB Cytochrome B-245 Beta Chain Protein Coding GSS Glutathione Synthetase Protein Coding CP Ceruloplasmin Protein Coding PRNP Prion Protein Protein Coding SLC11A2 Solute Carrier Family 11 Member 2 Protein Coding SLC40A1 Solute Carrier Family 40 Member 1 Protein Coding STEAP3 STEAP3 Metalloreductase Protein Coding ACSL1 Acyl-CoA Synthetase Long Chain Family Member 1 Protein -
Histone H3K9 Methylation Promotes Formation of Genome Compartments in Caenorhabditis Elegans Via Chromosome Compaction and Perinuclear Anchoring
Histone H3K9 methylation promotes formation of genome compartments in Caenorhabditis elegans via chromosome compaction and perinuclear anchoring Qian Biana,b,c,1, Erika C. Andersona,b,1,2, Qiming Yanga,b, and Barbara J. Meyera,b,3 aDepartment of Molecular and Cell Biology, University of California, Berkeley, CA 94720; bHoward Hughes Medical Institute, University of California, Berkeley, CA 94720; and cShanghai Institute of Precision Medicine, Ninth People’s Hospital, Shanghai Jiao Tong University School of Medicine, Shanghai, 200125, China Contributed by Barbara J. Meyer, March 17, 2020 (sent for review February 4, 2020; reviewed by Steven Henikoff and John Lis) Genomic regions preferentially associate with regions of similar mechanisms that establish them remain elusive. Most loops and transcriptional activity, partitioning genomes into active and inactive TADs can be eliminated by abrogating binding of key architectural compartments within the nucleus. Here we explore mechanisms con- proteins, including the SMC complex cohesin and the zinc-finger trolling genome compartment organization in Caenorhabditis ele- protein CTCF (16–19). However, genome compartments are gans and investigate roles for compartments in regulating gene preserved and enhanced in the absence of TADs, revealing that expression.DistalarmsofC. elegans chromosomes, which are compartments are created through different mechanisms (20). enriched for heterochromatic histone modifications H3K9me1/ The clustering of active and repressive genomic regions into sep- me2/me3, interact with each other both in cis and in trans, while arate compartments has been proposed to enhance transcriptional interacting less frequently with central regions, leading to genome regulation and stabilize different expression states. However, the compartmentalization. Arms are anchored to the nuclear periphery inability to perturb genome compartments has hindered our un- via the nuclear envelope protein CEC-4, which binds to H3K9me. -
Isolation, Characterization, and Expression in Normal and Tumor Cells1
(CANCER RESEARCH 58. 3401-3408, August I, I998| The Murine F hit Locus: Isolation, Characterization, and Expression in Normal and Tumor Cells1 Yuri Pekarsky,2 Teresa Druck,2 Maria Grazia Cotticelli, Masataka Ohta,3 Jiang Shou, Jeannine Menarola, Jeffry C. Montgomery, Arthur M. Buchberg, Linda D. Siracusa, Giacomo Manenti, Louise Y. Y. Fong, Tommaso A. Dragani, Carlo M. Croce, and Kay Huebner4 Kimmel Cancer Institute. Jefferson Medical College. Philadelphia. Pennsylvania 19107 [Y. P.. T. D.. M. G. C, M. O.. J. S.. J. M.. J. C. M.. A. M. B.. L D. S.. L Y. Y. F.. C. M. C.. K. H.¡;and Istituti! Na-ionale Tumori. Milan. Italy 20133 ¡C.M.. T. A. D.¡ ABSTRACT cancer cell lines and primary tumors, both normal and aberrant am plification products have been observed. Because the alterations to the The murine Fhit locus maps near the centromere v proximal Ptprg locus FHIT locus in many cancer cells appeared not to fit the classic tumor on mouse chromosome 14. The cDNA sequence and structure are similar suppressor paradigm, a number of investigators have suggested that to those of the human gene, with exons 5-9 encoding the protein. The the FHIT gene may not be a tumor suppressor gene. Alternative predominant mRNA in the tissues and cell lines tested was an alternatively spliced form missing exon 3. Most murine cell lines tested, including lines explanations for the very high frequency of alterations within the established from normal mouse embryos and tumors, expressed very low FHIT locus are: (a) the position of the FRA3B fragile region within or undetectable levels of Fhit mRNA. -
Antioxidants
antioxidants Article Short-Term Low Temperature Induces Nitro-Oxidative Stress that Deregulates the NADP-Malic Enzyme Function by Tyrosine Nitration in Arabidopsis thaliana Juan C. Begara-Morales 1 , Beatriz Sánchez-Calvo 1, María V. Gómez-Rodríguez 1, Mounira Chaki 1, Raquel Valderrama 1 , Capilla Mata-Pérez 1, Javier López-Jaramillo 2 , Francisco J. Corpas 3 and Juan B. Barroso 1,* 1 Group of Biochemistry and Cell Signaling in Nitric Oxide, Department of Experimental Biology, Center for Advanced Studies in Olive Grove and Olive Oils, Faculty of Experimental Sciences, University of Jaén, Campus “Las Lagunillas”, s/n, E-23071 Jaén, Spain; [email protected] (J.C.B.-M.); [email protected] (B.S.-C.); [email protected] (M.V.G.-R.); [email protected] (M.C.); [email protected] (R.V.); [email protected] (C.M.-P.) 2 Institute of Biotechnology, Department of Organic Chemistry, Faculty of Sciences, University of Granada, E-18071 Granada, Spain; [email protected] 3 Group of Antioxidants, Free Radicals, and Nitric Oxide in Biotechnology, Food and Agriculture, Department of Biochemistry, Cell and Molecular Biology of Plants, Estación Experimental del Zaidín, CSIC, C/Profesor Albareda 1, E-18080 Granada, Spain; [email protected] * Correspondence: [email protected] Received: 1 August 2019; Accepted: 20 September 2019; Published: 1 October 2019 Abstract: Low temperature (LT) negatively affects plant growth and development via the alteration of the metabolism of reactive oxygen and nitrogen species (ROS and RNS). Among RNS, tyrosine nitration, the addition of an NO2 group to a tyrosine residue, can modulate reduced nicotinamide-dinucleotide phosphate (NADPH)-generating systems and, therefore, can alter the levels of NADPH, a key cofactor in cellular redox homeostasis. -
Exome Sequencing and Human Disease: the Molecular
Exome Sequencing and Human Disease The molecular characterisation of genetic disorders by Diana Maria Walsh A thesis submitted to the University of Birmingham for the degree of DOCTOR OF PHILOSOPHY Institute of Biomedical Research School of Clinical and Experimental Medicine College of Medical and Dental Sciences University of Birmingham September 2015 0 University of Birmingham Research Archive e-theses repository This unpublished thesis/dissertation is copyright of the author and/or third parties. The intellectual property rights of the author or third parties in respect of this work are as defined by The Copyright Designs and Patents Act 1988 or as modified by any successor legislation. Any use made of information contained in this thesis/dissertation must be in accordance with that legislation and must be properly acknowledged. Further distribution or reproduction in any format is prohibited without the permission of the copyright holder. Abstract Since the completion of the human genome project in 2001, the field of genomics has advanced exponentially, largely in part to the introduction of next generation sequencing (NGS); a technique that has revolutionised the ways in which genetic disease is investigated. NGS enables the simultaneous sequencing of multiple reads in parallel, which provides researchers with the opportunity to interrogate vast numbers of candidate genes in order to establish the genetic eitiology and key components of disease. Exome sequencing in particular offers an efficient method to investigate disease, as the exomic regions make up 1% of the whole genome, but can contain up to 85% of functional variants responsible for disease. Next generation sequencing has been employed to investigate and identify the genetic cause of Acrocallosal syndrome (a rare autosomal recessive disorder).