Oxidation of Unactivated CH Bonds Catalyzed by Manganese Complexes
Total Page:16
File Type:pdf, Size:1020Kb
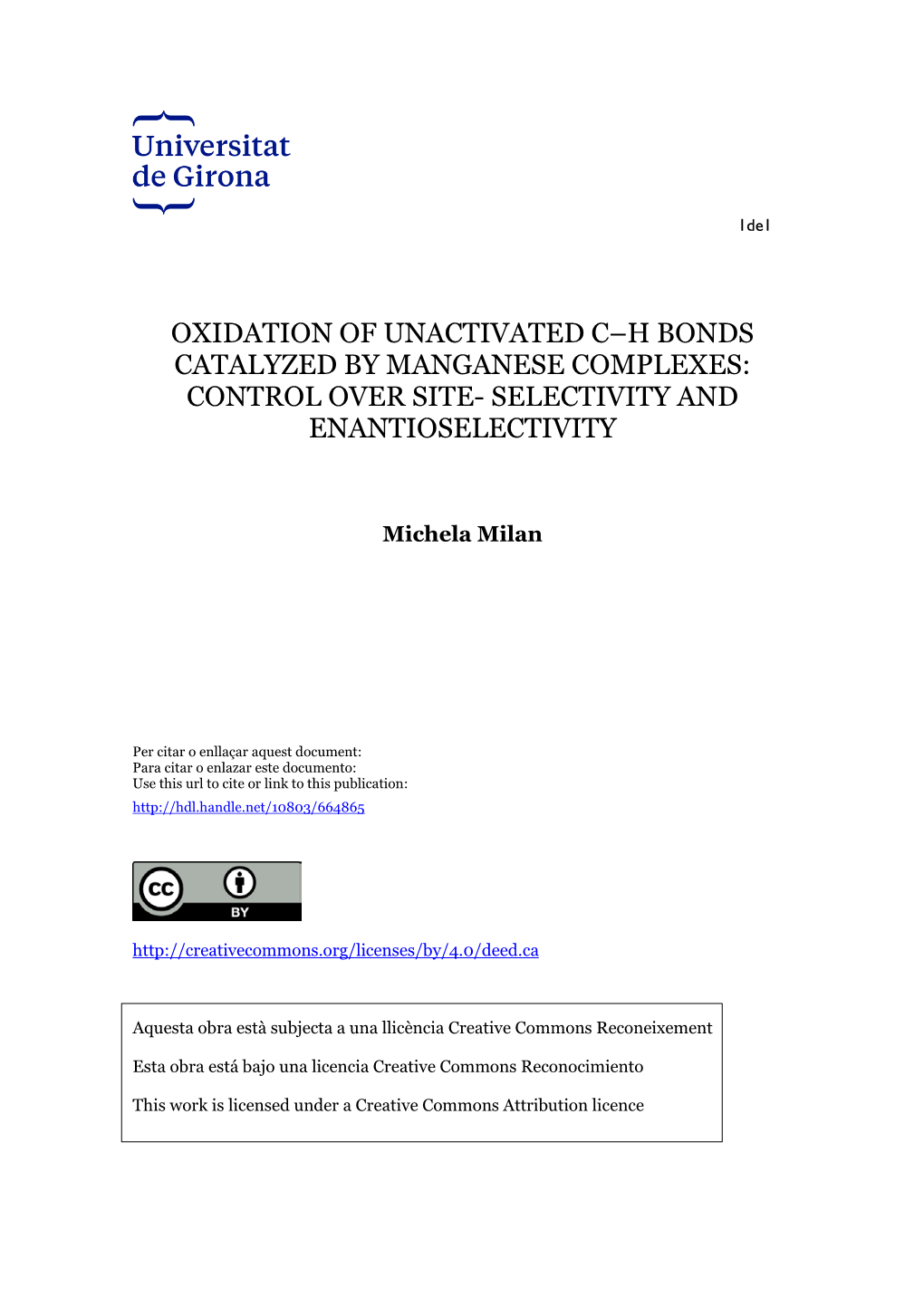
Load more
Recommended publications
-
The Oxidation of Secondary Alcohols by Dimethyldioxirane: Re-Examination of Kinetic Isotope Effects
Heterocycl. Commun., Vol. 16(4-6), pp. 217–220, 2010 • Copyright © by Walter de Gruyter • Berlin • New York. DOI 10.1515/HC.2010.016 Preliminary Communication The oxidation of secondary alcohols by dimethyldioxirane: re-examination of kinetic isotope effects A lfons L. Baumstark *, P edro C. Vasquez, Mark 1991 ; Denmark and Wu , 1998 ; Frohn et al. , 1998 ) or in an Cunningham a and Pamela M. Leggett-Robinson b isolated solution (Murray and Jeyaraman , 1985 ; Baumstark and McCloskey , 1987 ). The epoxidation of alkenes and het- Department of Chemistry , Center for Biotech and Drug eroatom oxidation by isolated solutions of 1 in acetone have Design, Georgia State University, Atlanta, Georgia been extensively investigated (Murray and Jeyaraman , 1985 ; 30302-4098 , USA Baumstark and McCloskey , 1987 ; Baumstark and Vasquez , * Corresponding author 1988 ; Winkeljohn et al. , 2004 , 2007 ). Dioxiranes can also e-mail: [email protected] insert oxygen into unactivated CH bonds of alkanes (Murray et al. , 1986 ). However, this important reaction generally requires a dioxirane more reactive than dimethyldioxirane to Abstract be of utility (Kuck et al. , 1994 ; D ’ Accolti et al., 2003 ). The oxidation of secondary alcohols by dimethyldioxirane, 1 , to The kinetic isotope effects for the oxidation of a series of ketones can be achieved in high yield under mild conditions deuterated isopropanols and α -trideuteromethyl benzyl alco- and with convenient reaction times (Kovac and Baumstark , hol by dimethyldioxirane ( 1 ) to the corresponding ketones 1994 ; Cunningham et al. , 1998; Baumstark, 1999 ). Two were determined in dried acetone at 23 ° C. A primary kinetic mechanistic extremes have been proposed for secondary alco- isotope effect (PKIE) of 5.2 for the oxidation of isopropyl- hol oxidation by 1 : a) concerted insertion (Mello et al. -
Chapter 13 Reactions of Arenes Electrophilic Aromatic Substitution
CH. 13 Chapter 13 Reactions of Arenes Electrophilic Aromatic Substitution Electrophiles add to aromatic rings in a fashion somewhat similar to the addition of electrophiles to alkenes. Recall: R3 R4 E Y E Y C C + E Y R1 C C R4 R1 C C R4 − R2 R1 δ+ δ R2 R3 R2 R3 In aromatic rings, however, we see substitution of one of the benzene ring hydrogens for an electrophile. H E + E Y + Y H δ+ δ− The mechanism is the same regardless of the electrophile. It involves two steps: (1) Addition of the electrophile to form a high-energy carbocation. (2) Elimination of the proton to restore the aromatic ring system. H H H H slow E Y + Y step 1 + E δ+ δ− high energy arenium ion H H step 2 fast E E + Y + Y H The first step is the slow step since the aromaticity of the benzene ring system is destroyed on formation of the arenium ion intermediate. This is a high energy species but it is stabilized by resonance with the remaining two double bonds. The second step is very fast since it restores the aromatic stabilization. 1 CH. 13 H H H H H H E E E There are five electrophilic aromatic substitution reactions that we will study. (1) Nitration H NO2 H2SO4 + HNO3 (2) Sulfonation H SO3H + H2SO4 (3) Halogenation with bromine or chlorine H X FeX3 X = Br, Cl + X2 (4) Friedel-Crafts Alkylation H R AlX + RX 3 (5) Friedel-Crafts Acylation O H O C AlX3 R + Cl C R 2 CH. -
Estimating the Densities of Benzene-Derived Explosives Using Atomic Volumes
Journal of Molecular Modeling (2018) 24: 50 https://doi.org/10.1007/s00894-018-3588-9 ORIGINAL PAPER Estimating the densities of benzene-derived explosives using atomic volumes Vikas D. Ghule1 & Ayushi Nirwan1 & Alka Devi1 Received: 7 November 2017 /Accepted: 8 January 2018 /Published online: 9 February 2018 # Springer-Verlag GmbH Germany, part of Springer Nature 2018 Abstract The application of average atomic volumes to predict the crystal densities of benzene-derived energetic compounds of general formula CaHbNcOd is presented, along with the reliability of this method. The densities of 119 neutral nitrobenzenes, energetic salts, and cocrystals with diverse compositions were estimated and compared with experimental data. Of the 74 nitrobenzenes for which direct comparisons could be made, the % error in the estimated density was within 0–3% for 54 compounds, 3–5% for 12 compounds, and 5–8% for the remaining 8 compounds. Among 45 energetic salts and cocrystals, the % error in the estimated density was within 0–3% for 25 compounds, 3–5% for 13 compounds, and 5–7.4% for 7 compounds. The absolute error surpassed 0.05 g/cm3 for 27 of the 119 compounds (22%). The largest errors occurred for compounds containing fused rings and for compounds with three –NH2 or –OH groups. Overall, the present approach for estimating the densities of benzene- derived explosives with different functional groups was found to be reliable. Keywords Density . Atomic volume . Explosive . Group additivity method . Energetic salts Introduction providing that the density and heat of formation values fed into the formula are reliable. The synthesis or hypothetical design of new explosive com- The purpose of the work reported in the present paper was to pounds requires the evaluation of various molecular and energet- develop a simple and straightforward correlation for predicting ic properties in order to select promising molecules. -
Functionalization of Heterocycles: a Metal Catalyzed Approach Via
FUNCTIONALIZATION OF HETEROCYCLES: A METAL CATALYZED APPROACH VIA ALLYLATION AND C-H ACTIVATION A Dissertation Submitted to the Graduate Faculty of the North Dakota State University of Agriculture and Applied Science By Sandeepreddy Vemula In Partial Fulfillment of the Requirements for the Degree of DOCTOR OF PHILOSOPHY Major Department: Chemistry and Biochemistry October 2018 Fargo, North Dakota North Dakota State University Graduate School Title FUNCTIONALIZATION OF HETEROCYCLES: A METAL CATALYZED APPROACH VIA ALLYLATION AND C-H ACTIVATION By Sandeepreddy Vemula The Supervisory Committee certifies that this disquisition complies with North Dakota State University’s regulations and meets the accepted standards for the degree of DOCTOR OF PHILOSOPHY SUPERVISORY COMMITTEE: Prof. Gregory R. Cook Chair Prof. Mukund P. Sibi Prof. Pinjing Zhao Prof. Dean Webster Approved: November 16, 2018 Prof. Gregory R. Cook Date Department Chair ABSTRACT The central core of many biologically active natural products and pharmaceuticals contain N-heterocycles, the installation of simple/complex functional groups using C-H/N-H functionalization methodologies has the potential to dramatically increase the efficiency of synthesis with respect to resources, time and overall steps to key intermediate/products. Transition metal-catalyzed functionalization of N-heterocycles proved as a powerful tool for the construction of C-C and C-heteroatom bonds. The work in this dissertation describes the development of palladium catalyzed allylation, and the transition metal catalyzed C-H activation for selective functionalization of electron deficient N-heterocycles. Chapter 1 A thorough study highlighting the important developments made in transition metal catalyzed approaches for C-C and C-X bond forming reactions is discussed with a focus on allylation, directed indole C-2 substitution and vinylic C-H activation. -
A Thiocyanopalladation/Carbocyclization Transformation Identified Through Enzymatic Screening
Chemical Science View Article Online EDGE ARTICLE View Journal | View Issue A thiocyanopalladation/carbocyclization transformation identified through enzymatic Cite this: Chem. Sci.,2017,8,8050 screening: stereocontrolled tandem C–SCN and C–C bond formation† G. Malik,‡ R. A. Swyka,‡ V. K. Tiwari, X. Fei, G. A. Applegate and D. B. Berkowitz * Herein we describe a formal thiocyanopalladation/carbocyclization transformation and its parametrization and optimization using a new elevated temperature plate-based version of our visual colorimetric enzymatic screening method for reaction discovery. The carbocyclization step leads to C–SCN bond formation in tandem with C–C bond construction and is highly stereoselective, showing nearly absolute 1,2-anti-stereoinduction (5 examples) for substrates bearing allylic substitution, and nearly absolute 1,3- syn-stereoinduction (16 examples) for substrates bearing propargylic substitution. Based upon these high levels of stereoinduction, the dependence of the 1,2-stereoinduction upon cyclization substrate Creative Commons Attribution-NonCommercial 3.0 Unported Licence. geometry, and the generally high preference for the transoid vinyl thiocyanate alkene geometry, a mechanistic model is proposed, involving (i) Pd(II)-enyne coordination, (ii) thiocyanopalladation, (iii) migratory insertion and (iv) b-elimination. Examples of transition metal-mediated C–SCN bond formation that proceed smoothly on unactivated substrates and allow for preservation of the SCN moiety are lacking. Yet, the thiocyanate functionality -
Fatty Acid Biosynthesis
BI/CH 422/622 ANABOLISM OUTLINE: Photosynthesis Carbon Assimilation – Calvin Cycle Carbohydrate Biosynthesis in Animals Gluconeogenesis Glycogen Synthesis Pentose-Phosphate Pathway Regulation of Carbohydrate Metabolism Anaplerotic reactions Biosynthesis of Fatty Acids and Lipids Fatty Acids contrasts Diversification of fatty acids location & transport Eicosanoids Synthesis Prostaglandins and Thromboxane acetyl-CoA carboxylase Triacylglycerides fatty acid synthase ACP priming Membrane lipids 4 steps Glycerophospholipids Control of fatty acid metabolism Sphingolipids Isoprene lipids: Cholesterol ANABOLISM II: Biosynthesis of Fatty Acids & Lipids 1 ANABOLISM II: Biosynthesis of Fatty Acids & Lipids 1. Biosynthesis of fatty acids 2. Regulation of fatty acid degradation and synthesis 3. Assembly of fatty acids into triacylglycerol and phospholipids 4. Metabolism of isoprenes a. Ketone bodies and Isoprene biosynthesis b. Isoprene polymerization i. Cholesterol ii. Steroids & other molecules iii. Regulation iv. Role of cholesterol in human disease ANABOLISM II: Biosynthesis of Fatty Acids & Lipids Lipid Fat Biosynthesis Catabolism Fatty Acid Fatty Acid Degradation Synthesis Ketone body Isoprene Utilization Biosynthesis 2 Catabolism Fatty Acid Biosynthesis Anabolism • Contrast with Sugars – Lipids have have hydro-carbons not carbo-hydrates – more reduced=more energy – Long-term storage vs short-term storage – Lipids are essential for structure in ALL organisms: membrane phospholipids • Catabolism of fatty acids –produces acetyl-CoA –produces reducing -
Synthesis of Oxymethylene Ethers
Synthesis of Oxymethylene Ethers Dissertation Zur Erlangung des akademischen Grades Doktor der Naturwissenschaften (Dr. rer. nat.) vorgelegt an der Fakultät für Chemie und Biochemie der Ruhr-Universität Bochum von Anna Grünert Bochum Oktober 2019 Die vorliegende Arbeit wurde in der Zeit von Februar 2016 bis Oktober 2019 in der Abteilung für Heterogene Katalyse am Max-Planck-Institut für Kohlenforschung in Mülheim an der Ruhr unter der Leitung von Prof. Dr. Ferdi Schüth angefertigt. Referent: Prof. Dr. Ferdi Schüth Korreferent: Prof. Dr. Martin Muhler I I Acknowledgements Firstly, I would like to thank my team of supervisors, Prof. Dr. Ferdi Schüth and Dr. Wolfgang Schmidt, and my co-examiner Prof. Dr. Martin Muhler. Ferdi, I would like to thank you for your trust that is the basis of the exceptional freedom of work, which you grant not only to me, but to all of your PhD students. You gave me the resources, time and liberty to develop my PhD project in my own way, to make mistakes, to solve challenging problems and to grow as a person. I am grateful for your appreciation of a cooperative and welcoming atmosphere in the group, which is most apparent in your yearly invitation to the group trip to Oberwesel. Wolfgang, I owe my thanks to you for your advice on many topics including material synthesis, catalyst characterisation and manuscript writing. I very much appreciate your welcoming and relaxed attitude. I am also thankful that to you both that you enjoy sharing your knowledge and experience with me and my colleagues in catalysis seminars and other technical seminars and in the focused project meetings. -
Ganic Compounds
6-1 SECTION 6 NOMENCLATURE AND STRUCTURE OF ORGANIC COMPOUNDS Many organic compounds have common names which have arisen historically, or have been given to them when the compound has been isolated from a natural product or first synthesised. As there are so many organic compounds chemists have developed rules for naming a compound systematically, so that it structure can be deduced from its name. This section introduces this systematic nomenclature, and the ways the structure of organic compounds can be depicted more simply than by full Lewis structures. The language is based on Latin, Greek and German in addition to English, so a classical education is beneficial for chemists! Greek and Latin prefixes play an important role in nomenclature: Greek Latin ½ hemi semi 1 mono uni 1½ sesqui 2 di bi 3 tri ter 4 tetra quadri 5 penta quinque 6 hexa sexi 7 hepta septi 8 octa octo 9 ennea nona 10 deca deci Organic compounds: Compounds containing the element carbon [e.g. methane, butanol]. (CO, CO2 and carbonates are classified as inorganic.) See page 1-4. Special characteristics of many organic compounds are chains or rings of carbon atoms bonded together, which provides the basis for naming, and the presence of many carbon- hydrogen bonds. The valency of carbon in organic compounds is 4. Hydrocarbons: Compounds containing only the elements C and H. Straight chain hydrocarbons are named according to the number of carbon atoms: CH4, methane; C2H6 or H3C-CH3, ethane; C3H8 or H3C-CH2-CH3, propane; C4H10 or H3C-CH2- CH2-CH3, butane; C5H12 or CH3CH2CH2CH2CH3, pentane; C6H14 or CH3(CH2)4CH3, hexane; C7H16, heptane; C8H18, octane; C9H20, nonane; C10H22, CH3(CH2)8CH3, decane. -
Comparative Responses of Rice (Oryza Sativa) Straw to Urea Supplementation and Urea Treatment
COMPARATIVE RESPONSES OF RICE (ORYZA SATIVA) STRAW TO UREA SUPPLEMENTATION AND UREA TREATMENT M. N. A. Kumar1, K. Sundareshan, E. G. Jagannath S. R. Sampath and P. T. Doyle2 Southern Regional Station, National Dairy Research Institute, Bangalore - 560030, India Summary Twenty five 75% Holstein Friesian cross bred bullocks fed rice straw (Oryza saliva) of long form, were fed with the following five treatments. 1. Rice straw, untreated (RS) 2. RS + water (1:1), stored for 24 hours (WRS) 3. RS (100 kg) + urea solution (4 kg urea/100 litre water) and dried (USRS) 4. RS (100 kg) + urea solution (as in 3) stored in wet condition for 24 hours (UWRS) 5. RS (100 kg) + urea solution (as in 3) stored in pit for 21 days (UTRS). Potential digestibility of treatments of RS was evaluated by monitoring (in vitro) Simulating Rumen like Fermentation (SRLF). The results indicated that Dry Matter Intake (DMI), digestibility of nutrients, N utilization were of the order UTRS > UWRS > USRS > WRS and RS (p < 0.05 to P < 0.01). SRLF index was high (255.84) for UTRS and least (145.58) for USRS. It was interm ediary (199.66) for UWRS. The acetyl content (AC) of UTRS with higher hemicellulose (HCE) dig estibility (80.8%) was low compared to UWRS, USRS, RS and WRS. The acetate content was of the order UTRS < UWRS < USRS < WRS and RS thereby indicating that reduction in acetyl con tent was an index of positive response of urea-treatment of RS. In addition, the ratio of HCE/AC in faeces of UTRS was 0.87 as against the ratios (2.26-2.48) observed in other treatments recording reduction jn AC due to urea-treatinent. -
Molecular Structure of Wlbb, a Bacterial N-Acetyltransferase Involved in the Biosynthesis †,‡ of 2,3-Diacetamido-2,3-Dideoxy-D-Mannuronic Acid James B
4644 Biochemistry 2010, 49, 4644–4653 DOI: 10.1021/bi1005738 Molecular Structure of WlbB, a Bacterial N-Acetyltransferase Involved in the Biosynthesis †,‡ of 2,3-Diacetamido-2,3-dideoxy-D-mannuronic Acid James B. Thoden and Hazel M. Holden* Department of Biochemistry, University of Wisconsin, Madison, Wisconsin 53706 Received April 14, 2010; Revised Manuscript Received April 29, 2010 ABSTRACT: The pathogenic bacteria Pseudomonas aeruginosa and Bordetella pertussis contain in their outer membranes the rare sugar 2,3-diacetamido-2,3-dideoxy-D-mannuronic acid. Five enzymes are required for the biosynthesis of this sugar starting from UDP-N-acetylglucosamine. One of these, referred to as WlbB, is an N-acetyltransferase that converts UDP-2-acetamido-3-amino-2,3-dideoxy-D-glucuronic acid (UDP- GlcNAc3NA) to UDP-2,3-diacetamido-2,3-dideoxy-D-glucuronic acid (UDP-GlcNAc3NAcA). Here we report the three-dimensional structure of WlbB from Bordetella petrii. For this analysis, two ternary structures were determined to 1.43 A˚resolution: one in which the protein was complexed with acetyl-CoA and UDP and the second in which the protein contained bound CoA and UDP-GlcNAc3NA. WlbB adopts a trimeric quaternary structure and belongs to the LβH superfamily of N-acyltransferases. Each subunit contains 27 β-strands, 23 of which form the canonical left-handed β-helix. There are only two hydrogen bonds that occur between the protein and the GlcNAc3NA moiety, one between Oδ1 of Asn 84 and the sugar C-30 amino group and the second between the backbone amide group of Arg 94 and the sugar C-50 carboxylate. -
Chemical Innovation Technologies to Make Processes and Products More Sustainable
United States Government Accountability Office Center for Science, Technology, and Engineering Natural Resources and Environment Report to Congressional Requesters February 2018 TECHNOLOGY ASSESSMENT Chemical Innovation Technologies to Make Processes and Products More Sustainable GAO-18-307 The cover image displays a word cloud generated from the transcript of the meeting we convened with 24 experts in the field of sustainable chemistry. The size of the words in the cloud corresponds to the frequency with which each word appeared in the transcript. In most cases, similar words—such as singular and plural versions of the same word— were combined into a single term. Words that were unrelated to the topic of sustainable chemistry were removed. The images around the periphery are stylized representations of chemical molecules that seek to illustrate a new conceptual framework, whereby molecules can be transformed to provide better performance; however, they are not intended to represent specific chemical compounds. TECHNOLOGY ASSESSMENT Highlights of GAO-18-307, a report to congressional requesters Chemical Innovation February 2018 Technologies to Make Processes and Products More Sustainable Why GAO did this study What GAO found Chemistry contributes to virtually every Stakeholders lack agreement on how to define sustainable chemistry and how to aspect of modern life and the chemical measure or assess the sustainability of chemical processes and products; these industry supports more than 25 percent differences hinder the development and adoption of more sustainable chemistry of the gross domestic product of the technologies. However, based on a review of the literature and stakeholder United States. While these are positive interviews, GAO identified several common themes underlying what sustainable contributions, chemical production can chemistry strives to achieve, including: have negative health and environmental · improve the efficiency with which natural resources—including energy, consequences. -
215-216 HH W12-Notes-Ch 15
Chem 215 F12 Notes Notes – Dr. Masato Koreeda - Page 1 of 17. Date: October 5, 2012 Chapter 15: Carboxylic Acids and Their Derivatives and 21.3 B, C/21.5 A “Acyl-Transfer Reactions” I. Introduction Examples: note: R could be "H" R Z R O H R O R' ester O carboxylic acid O O an acyl group bonded to R X R S acid halide* R' an electronegative atom (Z) thioester O X = halogen O R' R, R', R": alkyl, alkenyl, alkynyl, R O R' R N or aryl group R" amide O O O acid anhydride one of or both of R' and R" * acid halides could be "H" R F R Cl R Br R I O O O O acid fluoride acid chloride acid bromide acid iodide R Z sp2 hybridized; trigonal planar making it relatively "uncrowded" O The electronegative O atom polarizes the C=O group, making the C=O carbon "electrophilic." Resonance contribution by Z δ * R Z R Z R Z R Z C C C C O O O δ O hybrid structure The basicity and size of Z determine how much this resonance structure contributes to the hybrid. * The more basic Z is, the more it donates its electron pair, and the more resonance structure * contributes to the hybrid. similar basicity O R' Cl OH OR' NR'R" Trends in basicity: O weakest increasing basiciy strongest base base Check the pKa values of the conjugate acids of these bases. Chem 215 F12 Notes Notes –Dr. Masato Koreeda - Page 2 of 17.