Complete Thesis
Total Page:16
File Type:pdf, Size:1020Kb
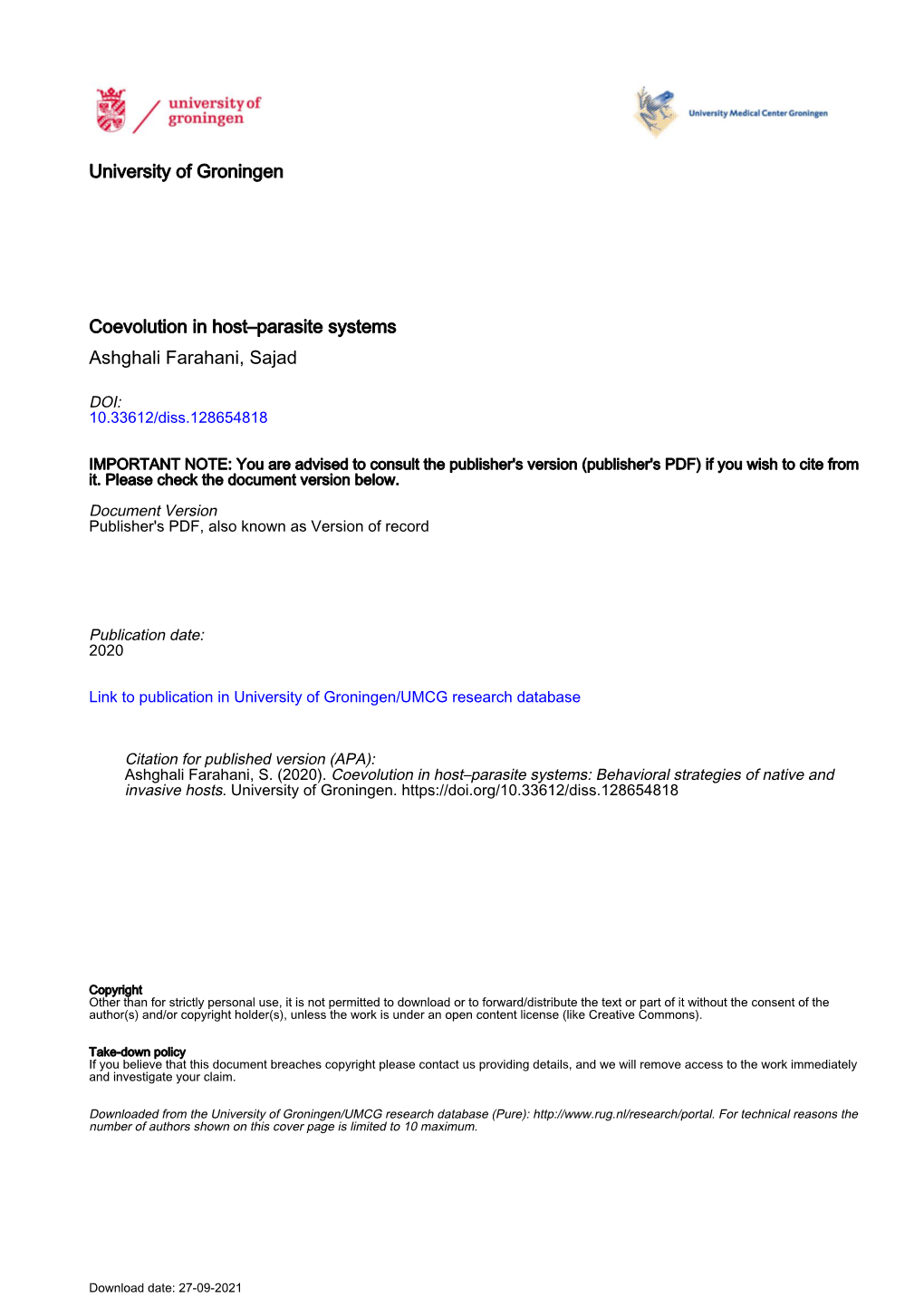
Load more
Recommended publications
-
Ansichten Der Altenau
Ansichten der Altenau Bilder von Michael Weber und Texte von Hartmut Lux 1 Dieses Buch entstand mit freundlicher Unterstützung durch: Ansichten der Altenau Selbstverlag Michael Weber, Grüner Weg 14, D-33178 Borchen-Nordborchen www.weber-bilder.de Erste Auflage im November 2004. Bildbearbeitung: RLS Jakobsmeyer GmbH. Druck: M.P. Media-Print Informationstechnologie GmbH. Alle Rechte vorbehalten. Copyrights © 2004 by Michael Weber. Bilder: Copyrights © 2004 by Michael Weber. Texte: Copyrights © 2004 by Hartmut Lux. Printed in Germany. 2 Meinem Neffen Max Weber (geb. am 6. Juni 2003) Dagegen aber behaupte ich, daß ein unmittelbares Interesse an der Schönheit der Natur zu nehmen (nicht bloß Geschmack haben, um sie zu beurteilen) jederzeit ein Kennzeichen einer guten Seele sei. Immanuel Kant: Kritik der Urteilskraft (1790), §42 3 Das Wasser der Altenau stürzt in Nordborchen, kurz vor der Mündung in die Alme, über einen Damm in die Lohne. 4 Vorwort Wer würde fotografieren – über einen Zeitraum von mehr als zehn Jahren immer wieder einen bestimmten Baum (Michael Weber: „Ansichten eines Kastanienbaums“) oder wie nun vorliegend: einen Fluss, die Altenau, in all ihren verschiedenen Erscheinungsformen - wenn er nicht im äußeren Anblick etwas erlebte, einen inneren Gehalt, mit dem man wie in ein Gespräch eintritt. Ebenso wird, wer Lyrik verfasst, zum Sinneseindruck, zur Sinneserfahrung (die wechselweise Bedeutung des Wörtchens ‚Sinn’ verwirklichend) eben diese ‚innere’, begrifflich-sinnhafte Seite zum Ausdruck bringen wollen. Michael Webers Fotografien mit lyrischen Beigaben zu Aus: „Ansichten eines Kastanienbaums“ versehen, war auch so verstanden eine sehr reiz– und anspruchsvolle Aufgabe. Mit den nun vorliegenden Zuordnungen ist versucht, einerseits den Fotografien nicht durch allzugroße Nähe den Raum zu nehmen, den sie als eigenständige Bildworte beanspruchen. -
Quellschwemmkegel – Eine Sonderform Temporärer Karst- Quellen Auf Der Paderborner Hochfläche
Stand: 2007 Quellschwemmkegel – eine Sonderform temporärer Karst- quellen auf der Paderborner Hochfläche Die Paderborner Hochfläche ist nicht Drainage ganz oder teilweise zerstört schwemmt und von unterirdischen Gebiet und Identität nur die größte, sondern auch die wasser- worden sind. Ende der 1990er Jahre Karstwasserströmen mitgeführt. Zudem reichste Karstlandschaft Westfalens wurden auch im Altenaugebiet vier werden bei der Lösung von anstehen- (vgl. Abb. 1). An ihrem Nordrand ent- QSK aufgefunden. Der größte von dem Gestein Residualtone freigesetzt, springen entlang der Bundesstraße 1 in ihnen liegt im Mental, einem linken wodurch der Suspensionsanteil der Bad Lippspringe, Paderborn, Salzkot- Nebental der Altenau das unterhalb von Karstwässer zusätzlich erhöht wird. ten-Upsprunge und Geseke zahlreiche Henglarn mündet. Ihm widmeten FEIGE Wasserdruck und Turbulenzen verhin- Dauerquellen, von denen allein die und OTTO 1999 in „GeKo aktuell“ eine dern weitgehend eine Klärung der Trübe Paderquellen 5 m3/s im Mittel schütten. erste Veröffentlichung. In den folgenden auf dem unterirdischen Lauf, und so Im Karstgebiet selbst finden sich dage- Jahren wurden die Untersuchungen am werden die mitgeführten Schwebstoffe Naturraum gen nur in der Nähe der tief liegenden Henglarner QSK fortgesetzt und auf in den Quellen zutage gefördert. Hier Konfluenz von Alme und Altenau bei weitere, insbesondere die Tudorfer erlischt die Transportkraft, und die Trü- Borchen einige wenige perennierende Quellen ausgedehnt (FEIGE/OTTO 2005). be wird ringförmig um die Quellöffnun- Quellen. Alle übrigen versiegen in den Die Entstehung der QSK lässt sich gen im Gras der Talauen abgelagert. Die Sommermonaten zeitweilig. Sie werden wie folgt erklären: Auf den offenen frischen Ablagerungen werden von die- im Paderborner Land Quickspringe ge- Feldfluren zwischen den Tälern wird in sem durchwachsen, und die QSK wer- nannt. -
Wasserverband Obere Lippe Stadt Paderborn
Wasserverband Obere Lippe Stadt Paderborn Umgestaltung des Ellerbaches in Paderborn - Ortsteil Dahl -Entwurf und Antrag auf Genehmigung gem. § 68 WHG- - Neufassung 2018 - Erläuterungsbericht Umgestaltung des Ellerbaches in Paderborn - Orts- teil Dahl -Entwurf und Antrag auf Genehmigung gem. § 68 WHG- - Neufassung 2018 - Mitwirkende: Detlef Sönnichsen Norbert Weinert Andreas Vetter Jan Oberdiek Johann Bruschinski © Eine Vervielfältigung oder Verwendung des Inhaltes in elektronischen oder gedruckten Publikationen aller Bestandteile dieses Berichts (inkl. Anlagen, digitalen Unterlagen etc.) ist ohne ausdrückliche vorhe- rige Zustimmung des Auftraggebers nicht gestattet. Z:\Auftg_15\A-36_15\Texte\A-36_15_WOL_Erl-Dahl_2018-05-22.docx Sönnichsen&Partner - Minden Umgestaltung des Ellerbaches in Paderborn - Ortsteil Dahl - Neufassung 2018 - 1 Inhalt 1 Veranlassung ............................................................................... 5 2 Grundlagen .................................................................................. 6 2.1 örtliche Überprüfungen .................................................................. 6 2.2 Datengrundlagen .......................................................................... 6 2.3 Software ....................................................................................... 7 3 Kurzbeschreibung der Örtlichkeit ................................................. 7 3.1 Hydrologie .................................................................................... 7 3.1.1 Bemessungsabflüsse ..................................................................... -
Die Altenau Soll Leben Ein Tal Bekommt Seinen Fluss Zurück
Die Altenau soll leben Ein Tal bekommt seinen Fluss zurück. MF/1 Die Altenau soll leben LEBENSADER ALTENAU IST VORBILDLICHES PROJEKT DIE ALTENAU SOLL LEBEN – NATURNÄHER GEWORDEN! MIT ENGAGIERTEN MENSCHEN SIE GEHT UNS ALLE AN Nach dem Hochwasserereignis 1965 wurden gro- Es ist außergewöhnlich, was im Altenautal im Früh- Die Altenau war schon immer ein wichtiger Be- ße Hochwasserschutzeinrichtungen im Altenautal jahr 2001 geschah. Die Menschen wollten ihren Fluss standteil unseres Tales, doch vor etwa 20 Jahren gebaut. zurück. Der Bach war ausgebaut und eing eengt, das bekam die Beziehung der Anrainer zu dem Fluss Die ökologischen Belange des Gewässers wur- wollten sie ändern. Die Altenau sollte wieder leben. schlagartig eine neue Qualität. Zu lange hatten wir den aber nicht ausreichend bedacht. Anlass für ein Auf Initiative des Attelner Heimatvereins forderten unseren Fluss genutzt, ohne Rücksicht auf Verluste. Umdenken war aber u.a. das zeitweilige Trockenfal- die Bürgerinnen und Bürger in einem Memorandum, Wir hatten die Altenau aus unserer Landschaft und len der Altenau, insbes. im Raum Atteln. Ich erinnere den Bach wieder natürlich zu gestalten. Sie warteten wohl auch aus unserem Bewusstsein weitgehend mich noch sehr genau daran, wie wir vor über 20 nicht auf den Staat und die Behörden. Sie wurden verdrängt. Die Quittung bekamen wir verblüffend Jahren fassungslos dieses Phänomen erstmals zur selbst aktiv und haben seither viel bewegt. Die Be- eindeutig präsentiert: der Fluss verschwand vor un- Kenntnis nehmen mussten. zirksregierung Detmold hat die Maßnahmen, die vor- seren Augen, er trocknete aus. Seitdem engagiert Im Jahr 2001 haben die Bürger des Altenautales bildlich vom Wasserverband Obere Lippe umgesetzt sich der Heimatverein Atteln intensiv und kontinuier- zwischen Blankenrode und Borchen unter Feder- wurden, bisher mit 1,3 Millionen Euro unterstützt. -
Broad-Scale Patterns of Invertebrate Richness and Community Composition
View metadata, citation and similar papers at core.ac.uk brought to you by CORE provided by Loughborough University Institutional Repository 1 Broad-scale patterns of invertebrate richness and community composition 2 in temporary rivers: effects of flow intermittence 3 T.Datry1, S.T. Larned2, K.M. Fritz3, M.T. Bogan4, P.J. Wood5, E.I. Meyer6, and A.N. Santos7 4 5 1 Institut national de Recherche en Sciences et Technologies pour l’Environnement et 6 l’Agriculture, Lyon, France, [email protected] 7 2 National Institute of Water and Atmospheric Research, Christchurch, New Zealand, 8 [email protected] 9 3 US-Environmental Protection Agency, Cincinnati, Ohio, USA, [email protected] 10 4 Oregon State University, Corvallis, Oregon, USA, [email protected] 11 5 Loughborough University, Leicestershire, UK, [email protected] 12 6 University of Münster, Münster, Germany, [email protected] 13 7 Texas A&M University, College Station, Texas, USA, [email protected] 14 15 Corresponding author: T. Datry, IRSTEA, UR MALY, 5 rue de la Doua, CS70077, 69926 16 Villeurbanne Cedex, France. Tel (33) 4.72.20.87.55 Fax (33) 4.78.47.78.75, 17 [email protected] 18 19 Running title: Invertebrate community patterns in temporary rivers 20 4 figures and 3 tables 21 2 Supplementary materials (S1, S2) 22 Number of references: 49 23 No. words: i. main text: 4006; ii. abstract: 251; iii. total including S1 and S2: 6943 24 25 1 26 Abstract (251 words) 27 Temporary rivers are increasingly common freshwater ecosystems, but there have been no 28 global syntheses of their community patterns. -
Steckbrief Sauer.Pdf
Stand: 26. Juni 2018 nrw.de - Gewässersteckbrief Sauer Wasserverband Obere Lippe Königsstraße 16, 33142 Büren Telefon (0 29 51) 9 33 90 - 0 Wasserverband Obere Lippe www.wol Obere 33142 Büren Wasserverband http://www.wol-nrw.de nrw.de - Inhaltsverzeichnis 1. Die Sauer – Steckbrief 2. Die Sauer – Kurzbeschreibung 3. Übersichtsplan 4. Historischer und heutiger Verlauf der Sauer 5. Preußische Uraufnahme 6. Naturräumliche Gliederung 7. Fließgewässertypen 7.1 Typ 7 Karstbach 7.2 Typ 11 Kleiner Talauenbach des Deckgebirges 9. Hydrologie und Klima 8. Geologie/Boden 9. Grundwasser 10. Fische 11. Vorher - Nachher Wasserverband Obere Lippe www.wol Obere 33142 Büren Wasserverband 1. Die Altenau – Steckbrief nrw.de Einzugsgebietsdaten: - Übersicht Einzugsgebietsdaten: Quelle: Lichtenau – Kleinenberg (367 mNHN) Mündung: Lichtenau – Atteln (189 mNHN) Fließlänge von der Quelle bis zur Mündung: 29,90 km Einzugsgebietsgröße Sauer: 110 km² Gewichtetes Sohlgefälle (gesamt): 5,95‰ relevante Zuflüsse im Verbandsgebiet: Bach von Kleinenberg bei GK 24+420 Odenheimer Bach bei GK 18+580 Schmittwasser bei GK 12+520 Gewässerkennzahlen: DE_NRW_278284_0 Fließgewässertyp: - von der Mündung Altenau bis zum HRB Sudheim: Karstbach (LAWA Typ 7) - vom HRB Sudheim bis zur Quelle: Kleiner Talauenbach des Deckgebirges Wasserverband Obere Lippe www.wol Obere 33142 Büren Wasserverband (LAWA Typ 11) Fischregionen: - von Mündung bis nrw.de Zufluss Odenheimer Bach: Karstbäche - - bis B 68 : oberer Forellentyp Karstbereiche - bis Quelle: oberer Forellentyp Mittelgebirge Mittlere Jahresniederschlagsmenge (Lichtenau): 901 mm Jahresdurchschnittstemperatur: 8,1 °C Abflussdaten HRB Sudheim: MNQ 0,150 m³/s MQ 0,400 m³/s HQ (1965) 56,00 m³/s Höhe der Quelle über NHN: 367 m Höhe der Mündung in die Alme über NHN: 189 m Durchschnittliche Wasserbreite (Mittellauf): - Ausbauzustand 8 – 12 m - naturnaher Zustand 6 – 8 m Durchschnittliche Wassertiefe (Mittellauf): - Ausbauzustand 1,5 – 2,5 m - naturnaher Zustand 0,5 – 1 m Wasserverband Obere Lippe www.wol Obere 33142 Büren Wasserverband 2. -
Borchen Stand März 2021
Hochwasserrisikomanagementplanung NRW Kommunensteckbrief Borchen Stand März 2021 Hochwasserrisikomanagementplanung in NRW Hochwasserrisiko und Maßnahmenplanung Borchen Die Karte zeigt die Risikogewässer und die Ausdehnung der Überflutung für das extreme Hochwasserereignis (HQextrem) im 2.Umsetzungszyklus 2016-2021 der HWRM-RL. Bezirksregierung Detmold Hochwasserrisikomanagementplanung NRW Kommunensteckbrief Borchen Stand März 2021 Der Kommunensteckbrief stellt die Maßnahmenplanung zur Verminderung von Hochwasserrisiken in Ihrer Kommune dar. Die Maßnahmenplanung ist ein wichtiger Schritt zur Umsetzung der europäischen Hochwas- serrisikomanagementrichtlinie (HWRM-RL) in Ihrer Region. Sie wurde auf der Grundlage der Hochwassergefahren- und Hochwasserrisikokarten für die Gewässer mit potenziellem signi- fikantem Hochwasserrisiko, die sogenannten Risikogewässer, erarbeitet. Mithilfe der Karten erkennen Sie, wo in Ihrer Region oder Ihrer Stadt konkret Gefahren und Risiken durch Hochwasser bestehen. Die aktuellen Gefahren- und Risikokarten und viele weitere Informationen zum Hochwasserrisikomanagement in NRW finden Sie auf der Inter- netseite flussgebiete.nrw.de oder in den Kartendiensten elwasweb.nrw.de bzw. uvo.nrw.de. Von welchen Risikogewässern ist Ihre Kommune betroffen? Teileinzugsgebiet (TEG) Lippe Flussgebiete NRW > TEG Lippe Alme Altenau Ellerbach Hinweis: Eine Hochwassergefährdung kann sich auch durch Gewässer ergeben, die hier nicht aufgeführt sind. Diese können in Ihrer Kommune liegen oder außerhalb. 2 Bezirksregierung Detmold -
Steckbrief Altenau.Pdf
Stand: 26. Juni 2018 nrw.de - Gewässersteckbrief Altenau Wasserverband Obere Lippe Königsstraße 16, 33142 Büren Telefon (0 29 51) 9 33 90 - 0 Wasserverband Obere Lippe www.wol Obere 33142 Büren Wasserverband http://www.wol-nrw.de nrw.de - Inhaltsverzeichnis 1. Die Altenau – Steckbrief 2. Die Altenau – Kurzbeschreibung 3. Übersichtsplan 4. Historischer und heutiger Verlauf der Altenau 5. Preußische Uraufnahme 6. Naturräumliche Gliederung 7. Fließgewässertypen 7.1 Typ 9.1 Schottergeprägter Karstfluss des Deckgebirges 7.2 Typ 7 Kastbach 9. Hydrologie und Klima 8. Geologie/Boden 9. Grundwasser 10. Fische 11. Vorher - Nachher Wasserverband Obere Lippe www.wol Obere 33142 Büren Wasserverband 1. Die Altenau – Steckbrief nrw.de Einzugsgebietsdaten: - Übersicht Einzugsgebietsdaten: Quelle: Lichtenau – Blankenrode (389 mNHN) Mündung: Nordborchen (126 mNHN) Fließlänge von der Quelle bis zur Mündung: 28,90 km Einzugsgebietsgröße Altenau: 335 km² Gewichtetes Sohlgefälle (gesamt): 9,1‰ relevante Zuflüsse im Verbandsgebiet: Holtheimer Bach bei GK 23+800 Piepenbach bei GK 20+270 Sauer bei GK 16+00 Ellerbach bei GK 1+800 Gewässerkennzahlen: DE_NRW_27828_0 (bis Mündung Sauer) DE_NRW_27828_16023 (bis Quelle) Fließgewässertyp: - von der Mündung Alme bis zur Mündung Sauer: schottergeprägter Karstfluss des Deckgebirges (LAWA Typ 9.1) - von der Mündung Sauer bis zur Quelle: Karstbach (LAWA Typ 7) Wasserverband Obere Lippe www.wol Obere 33142 Büren Wasserverband Hauptbodenart: Schluff bis Mittelsand, z.T. kalkhaltig oder mit Kies u. Steinen Fischregionen: - von Mündung bis Henglarn: Äschentyp Kartsbach nrw.de - bis Atteln: Karstfluss (keine Fischregion da - temporäres trockenfallen) - bis Zufluss Holtheimer Bach: oberer Forellentyp Karstbach - bis Quelle: oberer Forellentyp Mittelgebirge Mittlere Jahresniederschlagsmenge (Henglarn): 824 mm Jahresdurchschnittstemperatur: 8,8 °C Abflussdaten (Pegel Nordborchen): NQ 0,155 m³/s MNQ 0,480 m³/s MQ 2,06 m³/s MHQ 18,20 m³/s HQ 38,40 m³/s Anzahl Querbauwerke (inkl. -
CASE Study – Germany
CASE Study – Germany Altenau River Restoration of the Altenau River Technical Data (Source:WOL) Country Germany The Altenau River restoration project is a striking and Name of River Altenau / tributary of Alme educational example of several small dam removals and and Rhine dam conversions implemented in the frame of a river Name of Dam: Husen-Dalheim dam and restoration project. 51 weirs and ground sills The Altenau is a 28 kilometre long creek in the department Year of 1985: Husen-Dalheim dams Paderborn in Nord Rhine Westfalen Germany. During a construction 1965 -1985: weirs flood catastrophe in 1965 seven people died and a damage Year of 2002-2009: weirs and sills of several Million German Marks occurred. As a removal 2014-2017: partial dam consequence it was decided to regulate the creek and to removal of Husen-Dalheim construct several flood retention basins. In 1985 the dams Altenau was dammed and a large retention basin was Cost of 1,7 Mio. € for Husen-Dalheim created upstream the village Husen. removal: partial dam removal and restoration The intention of this 3ha large artificial lake was to promote Type of dam Flood protection regional tourism. Upstream this retention basin another Capacity - artificial lake was created for sediment disposal. Height / 4-5m Husen-Dalheim dams The environmental impacts of these artificial lakes were Length 0,5 -1,5m weirs and sills disastrous: In 1990 it was the first time as anyone can Volume - remember that the Altenau fell dry. It turned out, that Freed river km 45,8km (Altenau and about 80% of the impounded Altenau River was drained tributaries) into the underground beneath the artificial retention Dam owner: Wasserverband Obere Lippe basins, as these were situated above a karstic soil. -
Landschaftsplan Lichtenau Text.Pdf
KREIS PADERBORN Landschaftsplan Lichtenau Textliche Darstellungen und Festsetzungen mit Erläuterungen Erarbeitet von im Auftrag des Kreises Paderborn Landschaftsplan Lichtenau Seite I INHALTSVERZEICHNIS Vorwort ................................................................................................................ IV A. Planbestandteile, Verfahrenshinweise ............................................................. V B. Rechtsgrundlagen ............................................................................................. VII C. Räumlicher Geltungsbereich ........................................................................... VII D. Planerische Vorgaben und Grundlagen des Landschaftsplans .................. VII 1. Entwicklungsziele für die Landschaft ................................................................ 1 1.1 Entwicklungsziel 1 .................................................................................................. 2 1.2 Entwicklungsziel 2 .................................................................................................. 4 1.3 Entwicklungsziel 2a ................................................................................................ 5 1.4 Entwicklungsziel 3 .................................................................................................. 7 1.5 Entwicklungsziel 6 .................................................................................................. 7 2. Besonders geschützte Teile von Natur und Landschaft ............................... 10 2.1 -
Hochwasser-Gefahrenkarten Altenau (Mit Sauer, Schmittwasser, Odenheimer Bach)
Wasserverband Obere Lippe in Kooperation mit der Hochwasser-Gefahrenkarten Altenau (mit Sauer, Schmittwasser, Odenheimer Bach) für die beteiligten Städte und Gemeinden Borchen, Lichtenau Begleitheft Begleitheft-Gefahrenkarten-Altenau-2009-03- 10.doc Wasserverband Obere Lippe - Gefahrenkarten Altenau 1 Inhalt 1 Vorbemerkung......................................................................................................... 2 2 Information/Warnung der Bürger ............................................................................. 2 2.1 Grundsätze bei extremen Hochwasserfällen....................................................... 3 2.2 Aufgaben der Krisenstäbe ................................................................................. 3 2.3 Aufgaben der Einsatzkräfte................................................................................ 3 2.4 Aufgaben der Anwohner nach einem Ereignis.................................................... 4 3 Wellenlaufzeiten ...................................................................................................... 4 4 Straßensperren ........................................................................................................ 5 5 Datengrundlagen (Metadaten) ................................................................................. 6 5.1 Überschwemmungsflächen................................................................................ 6 5.2 Datengrundlagen.............................................................................................. 6 -
Die Altenau Soll Leben Ein Tal Bekommt Seinen Fluss Zurück
Die Altenau soll leben Ein Tal bekommt seinen Fluss zurück. MF/1 Die Altenau soll leben LEBENSadeR AlteNAU IST VORBIldlICHES PROJEKT DIE AlteNAU SOll leBEN – NatURNÄHER GEWORDEN! MIT ENGAGIERteN MENSCHEN SIE GEHT UNS ALLE AN Nach dem Hochwasserereignis 1965 wurden gro- Es ist außergewöhnlich, was im Altenautal im Früh- Die Altenau war schon immer ein wichtiger Be- ße Hochwasserschutzeinrichtungen im Altenautal jahr 2001 geschah. Die Menschen wollten ihren Fluss standteil unseres Tales, doch vor etwa 20 Jahren gebaut. zurück. Der Bach war ausgebaut und eingeengt, das bekam die Beziehung der Anrainer zu dem Fluss Die ökologischen Belange des Gewässers wur- wollten sie ändern. Die Altenau sollte wieder leben. schlagartig eine neue Qualität. Zulange hatten wir den aber nicht ausreichend bedacht. Anlass für ein Auf Initiative des Attelner Heimatvereins forderten unseren Fluss genutzt, ohne Rücksicht auf Verluste. Umdenken war aber u.a. das zeitweilige Trockenfal- die Bürgerinnen und Bürger in einem Memorandum, Wir hatten die Altenau aus unserer Landschaft und len der Altenau, insbes. im Raum Atteln. Ich erinnere den Bach wieder natürlich zu gestalten. Sie warteten wohl auch aus unserem Bewusstsein weitgehend mich noch sehr genau daran, wie wir vor über 20 nicht auf den Staat und die Behörden. Sie wurden verdrängt. Die Quittung bekamen wir verblüffend Jahren fassungslos dieses Phänomen erstmals zur selbst aktiv und haben seither viel bewegt. Die Be- eindeutig präsentiert: der Fluss verschwand vor un- Kenntnis nehmen mussten. zirksregierung Detmold hat die Maßnahmen, die vor- seren Augen, er trocknete aus. Seitdem engagiert Im Jahr 2001 haben die Bürger des Altenautales bildlich vom Wasserverband Obere Lippe umgesetzt sich der Heimatverein Atteln intensiv und kontinuier- zwischen Blankenrode und Borchen unter Feder- wurden, bisher mit 1,3 Millionen Euro unterstützt.