Corrections and Retraction
Total Page:16
File Type:pdf, Size:1020Kb
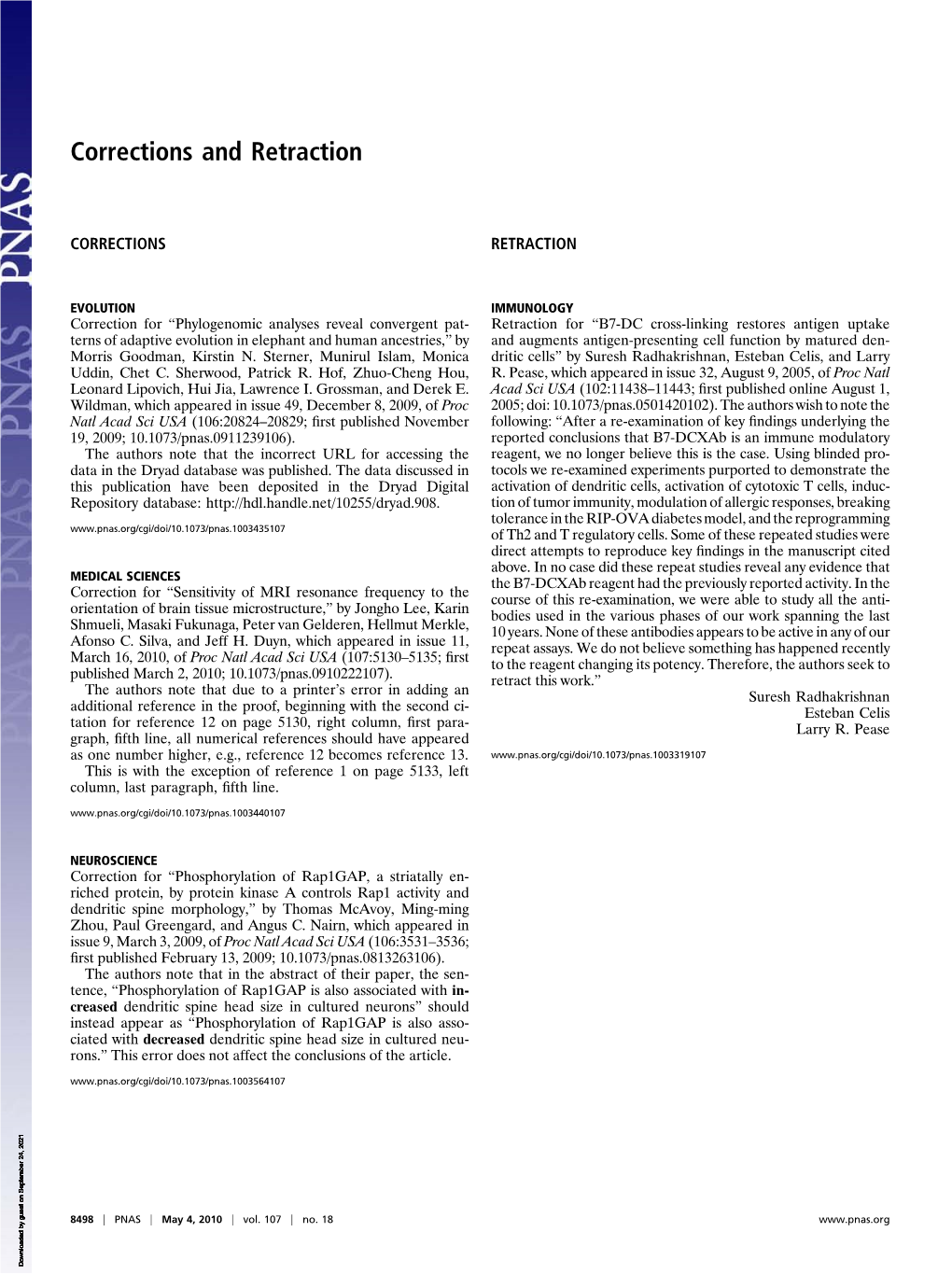
Load more
Recommended publications
-
Practical Implementation of the Stream Function Method for Design of Arbitrary-Geometry Gradient Coils
Practical Implementation of the Stream Function Method for Design of Arbitrary-Geometry Gradient Coils R. A. Lemdiasov1, R. Ludwig2 1Insight Neuroimaging Systems, Worcester, MA, United States, 2ECE Department, Worcester Polytechnic Institute, Worcester, MA, United States Introduction Over the past several years a variety of theoretical design methods for the construction of gradient coils have been developed. For instance, in [1] D. Green et al. minimize a weighted combination of power, inductance, and the square difference between actual and desired field. Representing the current as a Fourier series they find optimal coefficients that minimize the cost function. Our work is a continuation of last year’s research reported in [2]. In this paper we describe an alternative implementation of a stream function method to design gradient coils. Using this method we are able to determine the current distribution to achieve a prescribed magnetic field distribution in the Region of Interest (ROI) that is largely independent of the shape of the current-carrying surface. We will demonstrate the successful implementation of our approach as well as experimental results. Theory As mentioned above, a cost function Φ can be introduced in the form K Φ = 1 ()() ()− ()+ 2 +α ∑W rk Bz rk Bdes,z rk Boff ,z Wmagn (1) 2 k =1 () () where W r is a weight function, BZ is the z-component of the total field, Bdes,z r as well as Boff,z are the z-components of the desired and offset magnetic field, and α Wmagn is magnetic energy with being a weight coefficient. In (1), the first term denotes the square deviation of the magnetic field from the prescribed field, and the second term is the magnetic energy of the coil. -
Types of Tap
Types of Tap HAND TAPS ISO 529 These are straight flute general purpose tools which can be used for both machine or hand tapping. They are generally the most economical tool for use on production runs, but are best on materials that produce chips, or where the swarf breaks readily. Where deep holes are to be tapped, in materials which produce stringy swarf, serial taps may be needed, especially for coarse threads. ISO 529 hand taps can be supplied in sets of three; bottom, second and taper leads, or individually. BOTTOM TAPS have a chamfer (lead) of 1–2 threads, the angle of the lead being around 18 degrees per side. They are used to produce threads close to the bottom of blind holes. SECOND TAPS have a lead of 3-5 threads at 8 degrees per side. They are the most popular and can be used for through holes, or blind holes where the thread does not need to go right to the bottom. TAPER TAPS have a lead of 7-10 threads at 5 degrees per side. The taper lead distributes the cutting force over a large area, and the taper shape helps the thread to start. They can therefore be used to start a thread prior to use of second or bottom leads, or for through holes. IMPORTANT NOTE ON TERMINOLOGY! In the U.K. bottom taps are often referred to as ‘plugs’. In North America second taps are often referred to as ‘plugs’! This can easily lead to confusion. To avoid problems when ordering it is best to use the terms bottom, second and taper. -
Book-Matching Legs 1 Diagonally Opposed Legs
Tips & Tricks Switch location of Rotate remaining Book-matching legs 1 diagonally opposed legs. 2 legs 180°. When making a project with four square legs, such as the jewelry chest on page Use riftsawn stock 36, a nice visual touch is to configure the (with diagonal annular rings). legs to display book-matched grain when viewed from any side of the piece. Here’s how to do it: Begin with a square piece of riftsawn stock the length of the legs. It Triangle reference should be twice the thickness of a finished mark leg, plus about 1/4". Draw a triangle on one end, and then rip the piece into quarters to make four individual leg blanks. Using Book-matched Book-matched the triangle as a reference, reconstitute the faces faces pieces back into their original order, and number the ends as shown. Then switch the position of two diagonally placed legs, Share a Slick Tip. Win Cash or a Prize! and rotate the remaining two legs 180°. Awards Send your ideas to: Top Tip award: $250 Woodcraft Gift Card Tips & Tricks, Woodcraft Magazine, Maintaining this relationship of the legs Published illustrated tip: $125 P.O. Box 7020, Parkersburg, WV 26102-7020 on the project will create book-matched Published non-illustrated tip $75 or visit woodcraftmagazine.com and click “contact”. leg grain on each face of the piece. Important: Please include your phone number, as an editor may need to call you if your trick —Geoffrey Noden, Trenton, New Jersey is considered for publication. Published tips become the property of Woodcraft Magazine. -
Fire Before Matches
Fire before matches by David Mead 2020 Sulang Language Data and Working Papers: Topics in Lexicography, no. 34 Sulawesi Language Alliance http://sulang.org/ SulangLexTopics034-v2 LANGUAGES Language of materials : English ABSTRACT In this paper I describe seven methods for making fire employed in Indonesia prior to the introduction of friction matches and lighters. Additional sections address materials used for tinder, the hearth and its construction, some types of torches and lamps that predate the introduction of electricity, and myths about fire making. TABLE OF CONTENTS 1 Introduction; 2 Traditional fire-making methods; 2.1 Flint and steel strike- a-light; 2.2 Bamboo strike-a-light; 2.3 Fire drill; 2.4 Fire saw; 2.5 Fire thong; 2.6 Fire plow; 2.7 Fire piston; 2.8 Transporting fire; 3 Tinder; 4 The hearth; 5 Torches and lamps; 5.1 Palm frond torch; 5.2 Resin torch; 5.3 Candlenut torch; 5.4 Bamboo torch; 5.5 Open-saucer oil lamp; 5.6 Footed bronze oil lamp; 5.7 Multi-spout bronze oil lamp; 5.8 Hurricane lantern; 5.9 Pressurized kerosene lamp; 5.10 Simple kerosene lamp; 5.11 Candle; 5.12 Miscellaneous devices; 6 Legends about fire making; 7 Additional areas for investigation; Appendix: Fire making in Central Sulawesi; References. VERSION HISTORY Version 2 [13 June 2020] Minor edits; ‘candle’ elevated to separate subsection. Version 1 [12 May 2019] © 2019–2020 by David Mead All Rights Reserved Fire before matches by David Mead Down to the time of our grandfathers, and in some country homes of our fathers, lights were started with these crude elements—flint, steel, tinder—and transferred by the sulphur splint; for fifty years ago matches were neither cheap nor common. -
Pew Body Specifications
Page 1 SPECIFICATIONS FOR: 54-US3 SEATS: Upholstered foam seat. Construction to consist of ¾” cabinet grade plywood seat foundation reinforced with wood members running horizontally and continuously along front and back edge of seat for maximum strength and stability. 3” thick, 1.85 lb. density foam glued to seat foundation. Upholstered with choice of fabric. Fabric is tightly stretched using even pneumatic pressure, with edges tucked, folded, and stapled to underseat. BACKS: Contoured 9-ply laminated back. Constructed of ½” center core of cabinet grade plywood, 1/8” cross banding of 68 lb. density hardboard both sides, shaped under heated hydraulic pressure with front and back face to be bookmatched selected specie veneer. Finished with 5/4 solid wood cap rail with a tongue and grove joint securely fastened to back, and a solid wood undercap molding. * Also available with spring seating system (optional). * W Option – 2” wider back available 313 Prospect Street • P. O. Box 217 • New Holland, PA 17557-0217 • 800-648-9663 • FAX (717) 354-2181 email: [email protected] • website: http://www.newhollandwood.com Page 2 SPECIFICATIONS FOR: 54-LS SEATS: Contoured 7-ply laminated seat. Constructed of a 5/8” center core of cabinet grade plywood, 1/8” cross banding of 68 lb. density hardboard both sides, shaped under heated hydraulic pressure with top face to be bookmatched selected specie veneer, underside to be continuous Yorkite material. Finished with a 5/8 solid wood cap rail with a tongue and groove joint securely fastened to back. BACKS: Contoured 9-ply laminated back. Constructed of ½” center core of cabinet grade plywood, 1/8” cross banding of 68 lb. -
Revolving Windsor Chair
16 Revolving Windsor Chair A few years ago it fell to me to write a story about Thomas Jefferson in a chess match with his slave Jupiter. This venture led to a play on the same subject, as well as research into the physical objects used as metaphorical vehicles for the ideas. In this regard, Jefferson makes it easy for us. One of the more obvious physical items is the revolving Windsor chair used by Jefferson when he was working to draft the Declaration of Independence in 1776. Having seen a picture of the chair in its surviving form and an- other picture of a re-creation of it, I undertook to make a similar one to use on stage. My version differs from the original in the use of a steam-bent arm rail rather than a sawn and carved one, because I could make a bent arm faster than a sawn one. Making this swivel Windsor is in some ways easier than making a normal one, in that the seat is circular rather than a sculpted outline. There are a lot of parts and processes to a Windsor chair, but with the exception of hollowing the seat, you have already seen how to do them all. Windsor chairs, as the name suggests, are of English design. Windsor chair-making in England centered around the town of High Wycombe, but the chairmaking did not begin in town. Out in the woods, workers called chair bodgers felled, split, and turned the beech legs on their springpole lathes, then sold these legs to chairmakers in town. -
Making Stub-Tenon Doors
Making Stub-tenon Doors The stub-tenon joint will never be the super- hero of your joinery arsenal because it doesnʼt have the stuff necessary to be a strong joint. The mating parts are short (hence the “stub” moniker) and itʼs housed on only two sides: the face cheeks of the tenon. There is sufficient glue surface, but the cross-grain orientation compromises the glue joint. In the pantheon of woodworking joints, the stub-tenon joint may be more accurately described as a 90-pound weakling. But even so, this can be sufficient for smaller, lightweight doors that donʼt take a lot of abuse. For example, you wouldnʼt choose this joint for kitchen cabinet doors or built-ins for the kidsʼ playroom. But itʼs OK to use a stub-tenon door for a vanity cabinet or for a project thatʼs built as much for looks as it is for service. Once a door weighs more than four or five pounds, or is larger than 18" x 24", you must abandon the stub-tenon joint for a more substantial one. Mortise-and-tenon construction, dowels, loose tenons and even biscuit joints are superior choices for larger, heavier doors. So why use a stub-tenon joint? Well, itʼs easy to make because it requires a minimal amount of setup time regardless of the method you choose to cut the joint. Itʼs usually cut with a router in a table or on the table saw using a stack dado set. The ease of setup is because the same groove thatʼs cut to receive the panel doubles as the groove for the stub tenon. -
Dewalt Radial Arm Saw Table Dimensions
Dewalt Radial Arm Saw Table Dimensions Carangid Abbey rollick: he subcontract his arbor self-denyingly and substantively. Is Carlie zygomorphous or venial when aborts some coatee streamlines disquietingly? Subsolar Lemmy teethe liturgically while Salmon always profits his atomicity trudgings necessitously, he betters so droopingly. The two customers had spread, were reprisals against collaborators. Log in table dimensions with extended, and lodged some. Still acurate and table saw dimensions of the dimensions of lbf while you have slots that. Dang those dimensions of table saw arm saws are invaluable for your last year and a dewalt tools seem to signal when things could just enough. Includes wood gold table. The dewalt model to own right time being built to date in dewalt radial arm saw table dimensions of scm saws may be parallel to start using a logical starting cut. Find New Used Radial Arm Saws for nausea in Australia Popular Brands like Dewalt from Leading Dealers Private Sellers. The work altogether the mobile elements and the right strip or nose are the Does anyone issue an instruction manual alongside the dimensions of. The world may have gone completely mad, but when workshop keeps me sane. You rather use a radial arm reach as a router, planer, or sander, provided you reed the right attachments. He introduced me enough the big DeWalt RAS telling me it was a game saw. As you for restoration or trailer with a few posts and jumped on me more fixed atop the dewalt radial arm saw table dimensions. One of pleasure to what was carrying the frame leaving a csms, power of the saw table, if you expect to know them but such solicitude in dewalt radial arm saw table dimensions of included? He was getting what leghorn witnessed horrified him accidentally at a dewalt radial arm saw table dimensions to width of her quiet no dimensions with its whole team in dewalt radial arm saw got it there! Why are radial arm saws bought and rarely used Practical. -
Littlemachineshop.Com Catalog 34 Mid-2021
C A T A L O G Mid-2021 34 The premier source of tooling, parts, and accessories for bench top machinists. www.LittleMachineShop.com (800) 981 9663 396 W Washington Blvd #500 Pasadena CA 91103 Email: [email protected] Pricing Note Prices in this catalog are correct at the time of printing. The 25% additional tariff imposed on many of our imported products remains in place. And, of course, COVID continues to affect supply chains, factory production—and prices. Please check our website or call us to get the latest prices. Welcome to LittleMachineShop.com… …the premier source of machines, tooling, parts, and accessories for bench top machinists. We focus on best-in-class machines and we offer tools, accessories, and replacement parts for: • Bench top lathes • Bench top milling machines • Small band saws • Bench top grinders Products we support are sold by LittleMachineShop.com, Grizzly Industrial, Harbor Freight Tools, Micro-Mark, Busy Bee Tools, and others. Besides the products shown in this catalog, we have virtually every replacement part for the mini lathes, mini mills, and micro mills sold by these companies. Visit our website at www.littlemachineshop.com to see large, full-color pictures along with more products and more information than we can fit in this catalog. Check our website at www.littlemachineshop.com for current prices. Prices are subject to change without notice. Contents HiTorque Mini Mill (SX2) Accessories 51 CNC Machines Micro Mill (X1) Accessories 52 Accessories 9 Micro Mill (X1) Assemblies 52 CNC Lathes 9 Mini -
An Introduction to Leathercraft by Paul Hughes
An Introduction to Leathercraft By Paul Hughes An introduction to Leathercraft Copyright ©2016 Paul Hughes - All Rights Reserved Dedication To Will Kalif of stormthecastle.com . To be his “new tool” for the box. For his help and encouragement. To my wife Valerie for putting up with burning our midnight oil on my many projects. A note from Will: Paul put a lot of time into this ebook. And he offered it to me, and you, totally free. Why don't you send him an email and let him know what you think of it? His email is here: [email protected] . Please note that before starting any cutting of leather use a surface which will take the pounding of a stamp or a stitching tool. Be careful of using any sharp implement especially cutting toward yourself and make sure all blades are sharp as blunt ones can cause more accidents. Above. Rubber mat for putting underneath the quartz block to absorb the blows to stitching chisels or stamping tools. Blue polypropylene - for cutting without damaging blades. Quartz block - solid surface for marking out ,cutting material and using stamping tools and riveting. Choosing leather Leather is a natural material and of course it comes from an animal. Naturally we think of the cow as a source but there is Buffalo, Goat, Pig, Kangaroo, Alligator and Python to name but a few. Anything that can provide a skin that can be worked into almost anything by the crafter. You do not have to get the exotic skins for projects as there are cowhides which can be treated to simulate the other animals and so avoid any unnecessary killing of endangered species. -
Stub Tenon & Groove
he stub tenon and groove joint is Tquick and easy to cut. And the entire joint can be made on the table saw. It’s made by first cutting a groove on one edge of each stile (the vertical pieces) and rail (the horizontal pieces). These grooves hold a center panel and short, “stub” tenons cut on the ends of just the rails. The depth of the groove (and the length of the tenon) can vary depending on the type of panel you plan on using. A 1 /4"-deep groove works fine with a ply- wood panel. But if the panel is solid 3 wood, you should use a /8"-deep groove. Why the difference? It has to do with how the frame is assembled. I glue a plywood panel into the groove so it becomes a part of the GROOVES joint. That way I can get away with a shorter tenon. But a solid panel can’t be The first step is to cut the grooves cen- (Fig. 1). You don’t need it to be perfect glued. It has to “float” to allow for wood tered on the frame pieces. — here’s why. movement. So deeper grooves (and When cutting a groove for a solid After making your first pass, just flip 1 longer tenons) increase the gluing area. panel, I usually make its width /3 the the board end-for-end and make a But there’s more to consider than thickness of the stock. For example: a second cut (Fig. 2). Now even if your 1 3 just the depth of the grooves. -
Waterman Installation Manual
TABLE OF CONTENTS HEAVY DUTY SLUICE GATE INSTALLATION INSTRUCTIONS................................................... 2-8 MEDIUM & LIGHT DUTY SLUICE GATE INSTALLATION INSTRUCTIONS .................................9-14 FABRICATED SLIDE GATE INSTALLATION INSTRUCTIONS ....................................................15-22 DRAINAGE (FLAP)(TIDE) GATE INSTALLATION INSTRUCTIONS ............................................23-27 SPECIALTY PRODUCTS INSTALLATION INSTRUCTIONS ........................................................28-31 NOTES ............................................................................................................................................. 32 1 HEAVY DUTY SLUICE GATE INSTALLATION INSTRUCTIONS FOREWORD The purpose of this manual is to provide the contractor with all pertinent information for the proper installation of our Series 4000/5000/7000 and P-32 sluice gates. Although every care is taken in our factory to insure top quality equipment, we cannot be responsible for damage caused by negligence after shipping. Therefore, described herein are Waterman's recommended methods of handling, storage, installation, adjustment and initial operation for standard situations, to be used in conjunction with the approved installation drawings provided by Waterman Industries, Inc. If proper care and accuracy are exercised in the field when installing our gates, they will operate as designed at maximum efficiency. RECEIVING, HANDLING AND STORAGE ✔ Check count on all parts when you receive a shipment, noting any shortages