Behavioural Innovation and the Evolution of Cognition in Birds
Total Page:16
File Type:pdf, Size:1020Kb
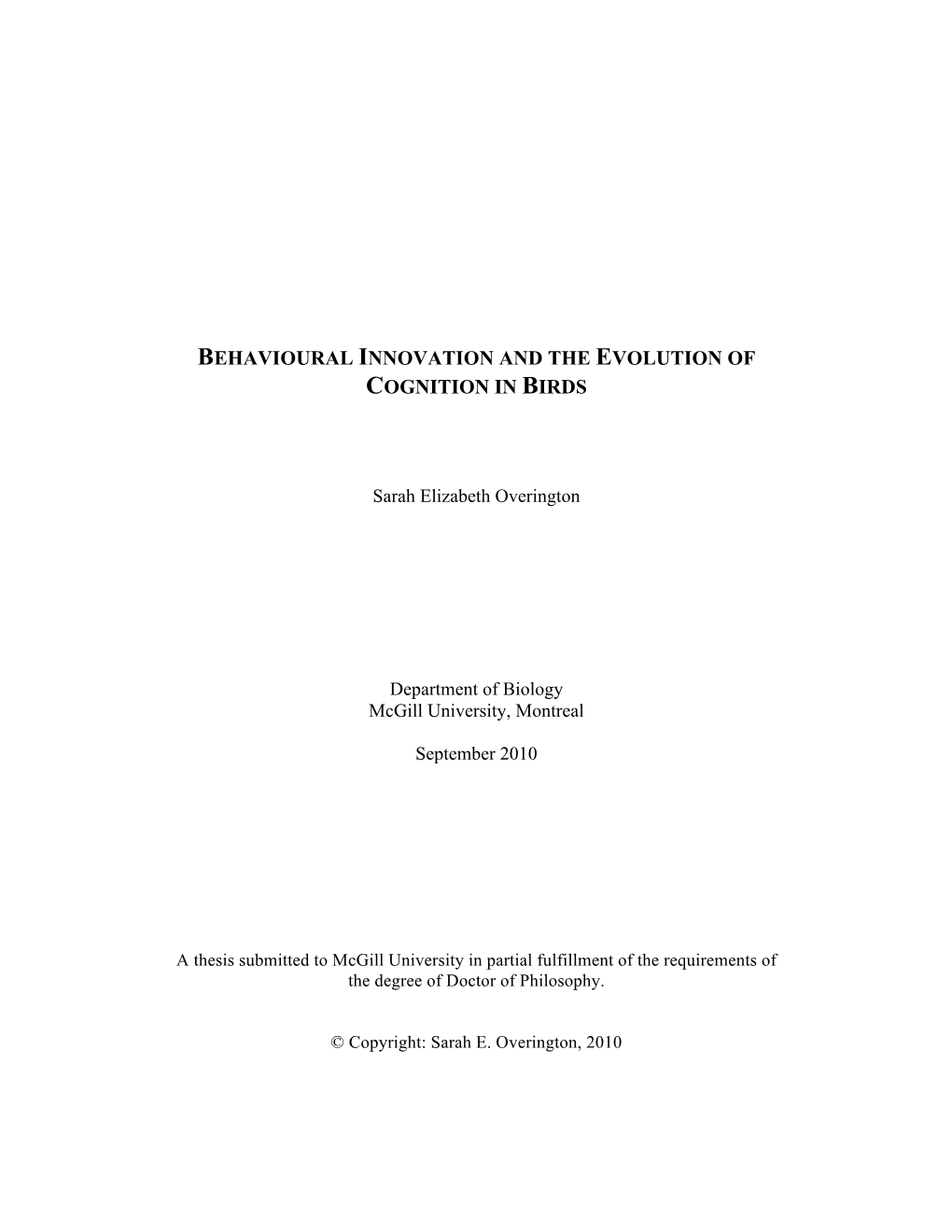
Load more
Recommended publications
-
Intelligence in Corvids and Apes: a Case of Convergent Evolution? Amanda Seed*, Nathan Emery & Nicola Claytonà
Ethology CURRENT ISSUES – PERSPECTIVES AND REVIEWS Intelligence in Corvids and Apes: A Case of Convergent Evolution? Amanda Seed*, Nathan Emery & Nicola Claytonà * Department of Psychology, Max Planck Institute for Evolutionary Anthropology, Leipzig, Germany School of Biological & Chemical Sciences, Queen Mary University of London, London, UK à Department of Experimental Psychology, University of Cambridge, Cambridge, UK (Invited Review) Correspondence Abstract Nicola Clayton, Department of Experimental Psychology, University of Cambridge, Downing Intelligence is suggested to have evolved in primates in response to com- Street, Cambridge CB23EB, UK. plexities in the environment faced by their ancestors. Corvids, a large- E-mail: [email protected] brained group of birds, have been suggested to have undergone a con- vergent evolution of intelligence [Emery & Clayton (2004) Science, Vol. Received: November 13, 2008 306, pp. 1903–1907]. Here we review evidence for the proposal from Initial acceptance: December 26, 2008 both ultimate and proximate perspectives. While we show that many of Final acceptance: February 15, 2009 (M. Taborsky) the proposed hypotheses for the evolutionary origin of great ape intelli- gence also apply to corvids, further study is needed to reveal the selec- doi: 10.1111/j.1439-0310.2009.01644.x tive pressures that resulted in the evolution of intelligent behaviour in both corvids and apes. For comparative proximate analyses we empha- size the need to be explicit about the level of analysis to reveal the type of convergence that has taken place. Although there is evidence that corvids and apes solve social and physical problems with similar speed and flexibility, there is a great deal more to be learned about the repre- sentations and algorithms underpinning these computations in both groups. -
Observation of Behavior, Inference of Function, and the Study of Learning
Psychonomic Bulletin & Review 1994, 1 (1), 73-88 Observation of behavior, inference of function, and the study of learning WILLIAM TIMBERLAKE and FRANCISCO J. SILVA Indiana University, Bloomington, Indiana Before the present century, the primary means of studying animals was observation of the form and effects of their behavior combined with presumption of their intent. In the present century, ethologists continued to emphasize observation of form and replaced presumption of intent with the study of proximate function and evolution. In contrast, most learning psychologists mini mized both observation of form and presumption of intent by defining behavior in terms of sim ple environmental effects and establishing intent by deprivation operations, We discuss advan tages of the use of observation in the study of learning, examine arguments that it is unnecessary, irrelevant, and unscientific, and consider some practical considerations in using observation. We conclude that observation of the form of behavior and concern with its ecological function should be an important part of the arsenal of techniques used to study learning. Observation has been the dominant method of study imals (Warden, 1927), anthropomorphically viewing the ing the behavior of animals since the beginning of re latter as partially disguised people enmeshed in a web of corded history (Warden, 1927). Observation provided the human goals, social relations, and rules (Aesop, approx underpinnings of the writings of systematists such as imately 620 B.C.; Selous, 1908). Other observers adhered Aristotle (384-322 B.C.) and Darwin (1859), story tellers to a more animal-centered description and inference of such as Seton (1913) and Kipling (1894), collectors of function (Craig, 1918; Huxley, 1914). -
Tinamiformes – Falconiformes
LIST OF THE 2,008 BIRD SPECIES (WITH SCIENTIFIC AND ENGLISH NAMES) KNOWN FROM THE A.O.U. CHECK-LIST AREA. Notes: "(A)" = accidental/casualin A.O.U. area; "(H)" -- recordedin A.O.U. area only from Hawaii; "(I)" = introducedinto A.O.U. area; "(N)" = has not bred in A.O.U. area but occursregularly as nonbreedingvisitor; "?" precedingname = extinct. TINAMIFORMES TINAMIDAE Tinamus major Great Tinamou. Nothocercusbonapartei Highland Tinamou. Crypturellus soui Little Tinamou. Crypturelluscinnamomeus Thicket Tinamou. Crypturellusboucardi Slaty-breastedTinamou. Crypturellus kerriae Choco Tinamou. GAVIIFORMES GAVIIDAE Gavia stellata Red-throated Loon. Gavia arctica Arctic Loon. Gavia pacifica Pacific Loon. Gavia immer Common Loon. Gavia adamsii Yellow-billed Loon. PODICIPEDIFORMES PODICIPEDIDAE Tachybaptusdominicus Least Grebe. Podilymbuspodiceps Pied-billed Grebe. ?Podilymbusgigas Atitlan Grebe. Podicepsauritus Horned Grebe. Podicepsgrisegena Red-neckedGrebe. Podicepsnigricollis Eared Grebe. Aechmophorusoccidentalis Western Grebe. Aechmophorusclarkii Clark's Grebe. PROCELLARIIFORMES DIOMEDEIDAE Thalassarchechlororhynchos Yellow-nosed Albatross. (A) Thalassarchecauta Shy Albatross.(A) Thalassarchemelanophris Black-browed Albatross. (A) Phoebetriapalpebrata Light-mantled Albatross. (A) Diomedea exulans WanderingAlbatross. (A) Phoebastriaimmutabilis Laysan Albatross. Phoebastrianigripes Black-lootedAlbatross. Phoebastriaalbatrus Short-tailedAlbatross. (N) PROCELLARIIDAE Fulmarus glacialis Northern Fulmar. Pterodroma neglecta KermadecPetrel. (A) Pterodroma -
REGUA Bird List July 2020.Xlsx
Birds of REGUA/Aves da REGUA Updated July 2020. The taxonomy and nomenclature follows the Comitê Brasileiro de Registros Ornitológicos (CBRO), Annotated checklist of the birds of Brazil by the Brazilian Ornithological Records Committee, updated June 2015 - based on the checklist of the South American Classification Committee (SACC). Atualizado julho de 2020. A taxonomia e nomenclatura seguem o Comitê Brasileiro de Registros Ornitológicos (CBRO), Lista anotada das aves do Brasil pelo Comitê Brasileiro de Registros Ornitológicos, atualizada em junho de 2015 - fundamentada na lista do Comitê de Classificação da América do Sul (SACC). -
Why Preen Others? Predictors of Allopreening in Parrots and Corvids and Comparisons to 2 Grooming in Great Apes
1 Why preen others? Predictors of allopreening in parrots and corvids and comparisons to 2 grooming in great apes 3 Short running title: Allopreening in parrots and corvids 4 Alejandra Morales Picard1,2, Roger Mundry3,4, Alice M. Auersperg3, Emily R. Boeving5, 5 Palmyre H. Boucherie6,7, Thomas Bugnyar8, Valérie Dufour9, Nathan J. Emery10, Ira G. 6 Federspiel8,11, Gyula K. Gajdon3, Jean-Pascal Guéry12, Matjaž Hegedič8, Lisa Horn8, Eithne 7 Kavanagh1, Megan L. Lambert1,3, Jorg J. M. Massen8,13, Michelle A. Rodrigues14, Martina 8 Schiestl15, Raoul Schwing3, Birgit Szabo8,16, Alex H. Taylor15, Jayden O. van Horik17, Auguste 9 M. P. von Bayern18, Amanda Seed19, Katie E. Slocombe1 10 1Department of Psychology, University of York, UK 11 2Montgomery College, Rockville, Maryland, USA 12 3Messerli Research Institute, University of Veterinary Medicine Vienna, Medical University 13 Vienna, University of Vienna, Vienna, Austria. 14 4Platform Bioinformatics and Biostatistics, University of Veterinary Medicine Vienna, Austria. 15 5Department of Psychology, Florida International University, Miami, FL, USA 16 6Institut Pluridisciplinaire Hubert Curien, University of Strasbourg, 23 rue Becquerel 67087 17 Strasbourg, France 18 7Centre National de la Recherche Scientifique, UMR 7178, 67087 Strasbourg, France 19 8Department of Cognitive Biology, University of Vienna, Vienna, Austria 20 9UMR Physiologie de la Reproduction et des Comportements, INRA-CNRS-Université de Tours 21 -IFCE, 37380 Nouzilly, France 22 10School of Biological & Chemical Sciences, Queen -
At Natural Foraging Sites Corvus Moneduloides Tool Use by Wild New Caledonian Crows
Downloaded from rspb.royalsocietypublishing.org on January 30, 2014 Tool use by wild New Caledonian crows Corvus moneduloides at natural foraging sites Lucas A. Bluff, Jolyon Troscianko, Alex A. S. Weir, Alex Kacelnik and Christian Rutz Proc. R. Soc. B 2010 277, doi: 10.1098/rspb.2009.1953 first published online 6 January 2010 Supplementary data "Data Supplement" http://rspb.royalsocietypublishing.org/content/suppl/2010/01/05/rspb.2009.1953.DC1.h tml References This article cites 23 articles, 5 of which can be accessed free http://rspb.royalsocietypublishing.org/content/277/1686/1377.full.html#ref-list-1 Article cited in: http://rspb.royalsocietypublishing.org/content/277/1686/1377.full.html#related-urls Subject collections Articles on similar topics can be found in the following collections behaviour (1094 articles) Receive free email alerts when new articles cite this article - sign up in the box at the top Email alerting service right-hand corner of the article or click here To subscribe to Proc. R. Soc. B go to: http://rspb.royalsocietypublishing.org/subscriptions Downloaded from rspb.royalsocietypublishing.org on January 30, 2014 Proc. R. Soc. B (2010) 277, 1377–1385 doi:10.1098/rspb.2009.1953 Published online 6 January 2010 Tool use by wild New Caledonian crows Corvus moneduloides at natural foraging sites Lucas A. Bluff1,*,†,‡, Jolyon Troscianko2, Alex A. S. Weir1, Alex Kacelnik1 and Christian Rutz1,*,‡ 1Department of Zoology, University of Oxford, South Parks Road, Oxford OX1 3PS, UK 2School of Biosciences, University of Birmingham, Birmingham B15 2TT, UK New Caledonian crows Corvus moneduloides use tools made from sticks or leaf stems to ‘fish’ woodboring beetle larvae from their burrows in decaying wood. -
Guia Para Observação Das Aves Do Parque Nacional De Brasília
See discussions, stats, and author profiles for this publication at: https://www.researchgate.net/publication/234145690 Guia para observação das aves do Parque Nacional de Brasília Book · January 2011 CITATIONS READS 0 629 4 authors, including: Mieko Kanegae Fernando Lima Favaro Federal University of Rio de Janeiro Instituto Chico Mendes de Conservação da Bi… 7 PUBLICATIONS 74 CITATIONS 17 PUBLICATIONS 69 CITATIONS SEE PROFILE SEE PROFILE All content following this page was uploaded by Fernando Lima Favaro on 28 May 2014. The user has requested enhancement of the downloaded file. Brasília - 2011 GUIA PARA OBSERVAÇÃO DAS AVES DO PARQUE NACIONAL DE BRASÍLIA Aílton C. de Oliveira Mieko Ferreira Kanegae Marina Faria do Amaral Fernando de Lima Favaro Fotografia de Aves Marcelo Pontes Monteiro Nélio dos Santos Paulo André Lima Borges Brasília, 2011 GUIA PARA OBSERVAÇÃO DAS AVES DO APRESENTAÇÃO PARQUE NACIONAL DE BRASÍLIA É com grande satisfação que apresento o Guia para Observação REPÚblica FEDERATiva DO BRASIL das Aves do Parque Nacional de Brasília, o qual representa um importante instrumento auxiliar para os observadores de aves que frequentam ou que Presidente frequentarão o Parque, para fins de lazer (birdwatching), pesquisas científicas, Dilma Roussef treinamentos ou em atividades de educação ambiental. Este é mais um resultado do trabalho do Centro Nacional de Pesquisa e Vice-Presidente Conservação de Aves Silvestres - CEMAVE, unidade descentralizada do Instituto Michel Temer Chico Mendes de Conservação da Biodiversidade (ICMBio) e vinculada à Diretoria de Conservação da Biodiversidade. O Centro tem como missão Ministério do Meio Ambiente - MMA subsidiar a conservação das aves brasileiras e dos ambientes dos quais elas Izabella Mônica Vieira Teixeira dependem. -
The Role of Agriculture in Avian Conservation in the Taita Hills, Kenya
This is a repository copy of Birds in the matrix : The role of agriculture in avian conservation in the Taita Hills, Kenya. White Rose Research Online URL for this paper: https://eprints.whiterose.ac.uk/129736/ Version: Accepted Version Article: Norfolk, Olivia, Jung, Martin, Platts, Philip J. orcid.org/0000-0002-0153-0121 et al. (3 more authors) (2017) Birds in the matrix : The role of agriculture in avian conservation in the Taita Hills, Kenya. African Journal of Ecology. pp. 530-540. ISSN 0141-6707 https://doi.org/10.1111/aje.12383 Reuse Items deposited in White Rose Research Online are protected by copyright, with all rights reserved unless indicated otherwise. They may be downloaded and/or printed for private study, or other acts as permitted by national copyright laws. The publisher or other rights holders may allow further reproduction and re-use of the full text version. This is indicated by the licence information on the White Rose Research Online record for the item. Takedown If you consider content in White Rose Research Online to be in breach of UK law, please notify us by emailing [email protected] including the URL of the record and the reason for the withdrawal request. [email protected] https://eprints.whiterose.ac.uk/ Birds in the matrix: the role of agriculture in avian conservation in the Taita Hills, Kenya Norfolk, O.1,2, Jung, M.3, Platts, P.J.4, Malaki, P.5, Odeny, D6. and Marchant, R.1 1. York Institute for Tropical Ecosystems, Environment Department, University of York, Heslington, York, UK, YO10 5NG 2. -
A Comprehensive Species-Level Molecular Phylogeny of the New World
YMPEV 4758 No. of Pages 19, Model 5G 2 December 2013 Molecular Phylogenetics and Evolution xxx (2013) xxx–xxx 1 Contents lists available at ScienceDirect Molecular Phylogenetics and Evolution journal homepage: www.elsevier.com/locate/ympev 5 6 3 A comprehensive species-level molecular phylogeny of the New World 4 blackbirds (Icteridae) a,⇑ a a b c d 7 Q1 Alexis F.L.A. Powell , F. Keith Barker , Scott M. Lanyon , Kevin J. Burns , John Klicka , Irby J. Lovette 8 a Department of Ecology, Evolution and Behavior, and Bell Museum of Natural History, University of Minnesota, 100 Ecology Building, 1987 Upper Buford Circle, St. Paul, MN 9 55108, USA 10 b Department of Biology, San Diego State University, San Diego, CA 92182, USA 11 c Barrick Museum of Natural History, University of Nevada, Las Vegas, NV 89154, USA 12 d Fuller Evolutionary Biology Program, Cornell Lab of Ornithology, Cornell University, 159 Sapsucker Woods Road, Ithaca, NY 14950, USA 1314 15 article info abstract 3117 18 Article history: The New World blackbirds (Icteridae) are among the best known songbirds, serving as a model clade in 32 19 Received 5 June 2013 comparative studies of morphological, ecological, and behavioral trait evolution. Despite wide interest in 33 20 Revised 11 November 2013 the group, as yet no analysis of blackbird relationships has achieved comprehensive species-level sam- 34 21 Accepted 18 November 2013 pling or found robust support for most intergeneric relationships. Using mitochondrial gene sequences 35 22 Available online xxxx from all 108 currently recognized species and six additional distinct lineages, together with strategic 36 sampling of four nuclear loci and whole mitochondrial genomes, we were able to resolve most relation- 37 23 Keywords: ships with high confidence. -
Naturalizing Anthropomorphism: Behavioral Prompts to Our Humanizing of Animals
WellBeing International WBI Studies Repository 2007 Naturalizing Anthropomorphism: Behavioral Prompts to Our Humanizing of Animals Alexandra C. Horowitz Barnard College Marc Bekoff University of Colorado Follow this and additional works at: https://www.wellbeingintlstudiesrepository.org/acwp_habr Part of the Animal Studies Commons, Comparative Psychology Commons, and the Other Anthropology Commons Recommended Citation Horowitz, A. C., & Bekoff, M. (2007). Naturalizing anthropomorphism: Behavioral prompts to our humanizing of animals. Anthrozoös, 20(1), 23-35. This material is brought to you for free and open access by WellBeing International. It has been accepted for inclusion by an authorized administrator of the WBI Studies Repository. For more information, please contact [email protected]. Naturalizing Anthropomorphism: Behavioral Prompts to Our Humanizing of Animals Alexandra C. Horowitz1 and Marc Bekoff2 1 Barnard College 2 University of Colorado – Boulder KEYWORDS anthropomorphism, attention, cognitive ethology, dogs, humanizing animals, social play ABSTRACT Anthropomorphism is the use of human characteristics to describe or explain nonhuman animals. In the present paper, we propose a model for a unified study of such anthropomorphizing. We bring together previously disparate accounts of why and how we anthropomorphize and suggest a means to analyze anthropomorphizing behavior itself. We introduce an analysis of bouts of dyadic play between humans and a heavily anthropomorphized animal, the domestic dog. Four distinct patterns of social interaction recur in successful dog–human play: directed responses by one player to the other, indications of intent, mutual behaviors, and contingent activity. These findings serve as a preliminary answer to the question, “What behaviors prompt anthropomorphisms?” An analysis of anthropomorphizing is potentially useful in establishing a scientific basis for this behavior, in explaining its endurance, in the design of “lifelike” robots, and in the analysis of human interaction. -
21 Sep 2018 Lists of Victims and Hosts of the Parasitic
version: 21 Sep 2018 Lists of victims and hosts of the parasitic cowbirds (Molothrus). Peter E. Lowther, Field Museum Brood parasitism is an awkward term to describe an interaction between two species in which, as in predator-prey relationships, one species gains at the expense of the other. Brood parasites "prey" upon parental care. Victimized species usually have reduced breeding success, partly because of the additional cost of caring for alien eggs and young, and partly because of the behavior of brood parasites (both adults and young) which may directly and adversely affect the survival of the victim's own eggs or young. About 1% of all bird species, among 7 families, are brood parasites. The 5 species of brood parasitic “cowbirds” are currently all treated as members of the genus Molothrus. Host selection is an active process. Not all species co-occurring with brood parasites are equally likely to be selected nor are they of equal quality as hosts. Rather, to varying degrees, brood parasites are specialized for certain categories of hosts. Brood parasites may rely on a single host species to rear their young or may distribute their eggs among many species, seemingly without regard to any characteristics of potential hosts. Lists of species are not the best means to describe interactions between a brood parasitic species and its hosts. Such lists do not necessarily reflect the taxonomy used by the brood parasites themselves nor do they accurately reflect the complex interactions within bird communities (see Ortega 1998: 183-184). Host lists do, however, offer some insight into the process of host selection and do emphasize the wide variety of features than can impact on host selection. -
Fraga Ms-706.Fm
View metadata, citation and similar papers at core.ac.uk brought to you by CORE provided by CONICET Digital SHORT COMMUNICATIONS ORNITOLOGIA NEOTROPICAL 19: 299–303, 2008 © The Neotropical Ornithological Society NOTES ON THE NESTING OF CHOPI BLACKBIRDS (GNORIMOPSAR CHOPI) IN ARGENTINA AND PARAGUAY, WITH DATA ON COOPERATIVE BREEDING AND BROOD PARASITISM BY SCREAMING COWBIRDS (MOLOTHRUS RUFOAXILLARIS) Rosendo M. Fraga CICYTTP, Consejo Nacional de Investigaciones Científicas y Técnicas (CONICET), Matteri y España, (3105) Diamante, Entre Ríos, Argentina. E-mail: [email protected] Notas sobre la nidificación del Chopí (Gnorimopsar chopi) en Argentina y Paraguay, con datos de cría cooperativa y de parasitismo de cría por el Tordo Pico Corto (Molothrus rufoaxillaris). Key words: Chopi Blackbirds, Gnorimopsar chopi, nesting, cooperative breeding, Screaming Cowbird, Molothrus rufoaxillaris. The Chopi Blackbird (Gnorimopsar chopi) is an northeastern Argentina (Corrientes and Mis- icterid endemic to South America (Jaramillo iones provinces) and Paraguay, between 1995 & Burke 1999) and a member of the South and 2007. My data show this icterid to be a American quiscaline clade, a monophyletic cooperative breeder (Brown 1987), as individ- group defined by DNA data (Johnson & uals other than a single pair aid in chick care. Lanyon 1999, Cadena et al. 2004). This icterid Also, I add further information on its host- is found from northeastern Brazil and south- parasite interactions with Screaming Cow- eastern Peru to northern Argentina and Uru- birds (Molothrus rufoaxillaris) in Argentina and guay (Jaramillo & Burke 1999). It is usually Paraguay (Sick 1985, Fraga 1996). subdivided into three subspecies with consid- I obtained most of my information on erable variation in body size (Jaramillo & Chopi Blackbirds while carrying field research Burke 1999), but only the nominate subspe- on endangered grassland birds from eastern cies occurs in eastern Argentina and Paraguay.