Space Weather – Impacts, Mitigation and Forecasting
Total Page:16
File Type:pdf, Size:1020Kb
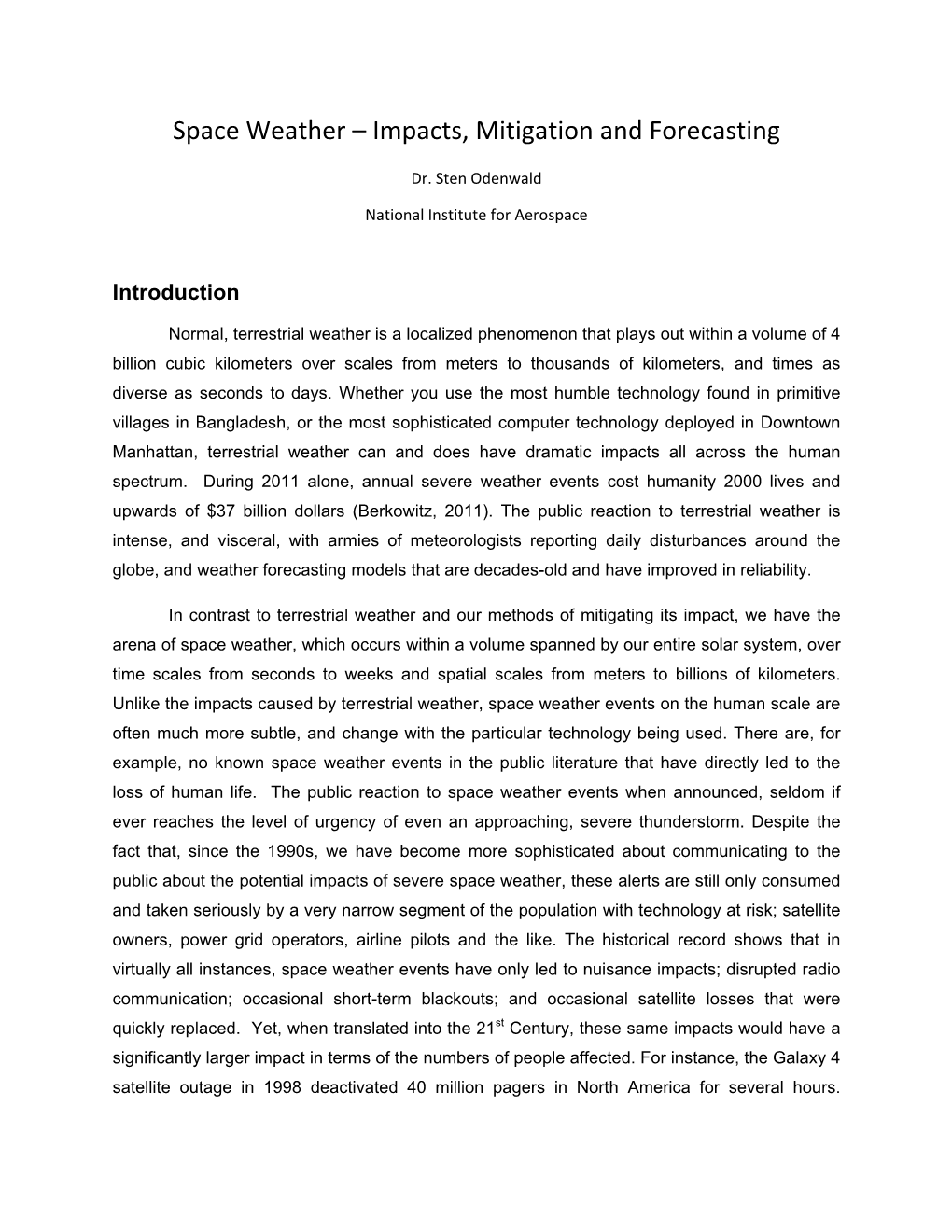
Load more
Recommended publications
-
Executive Summary
The Boston University Astronomy Department Annual Report 2010 Chair: James Jackson Administrator: Laura Wipf 1 2 TABLE OF CONTENTS Executive Summary 5 Faculty and Staff 5 Teaching 6 Undergraduate Programs 6 Observatory and Facilities 8 Graduate Program 9 Colloquium Series 10 Alumni Affairs/Public Outreach 10 Research 11 Funding 12 Future Plans/Departmental Needs 13 APPENDIX A: Faculty, Staff, and Graduate Students 16 APPENDIX B: 2009/2010 Astronomy Graduates 18 APPENDIX C: Seminar Series 19 APPENDIX D: Sponsored Project Funding 21 APPENDIX E: Accounts Income Expenditures 25 APPENDIX F: Publications 27 Cover photo: An ultraviolet image of Saturn taken by Prof. John Clarke and his group using the Hubble Space Telescope. The oval ribbons toward the top and bottom of the image shows the location of auroral activity near Saturn’s poles. This activity is analogous to Earth’s aurora borealis and aurora australis, the so-called “northern” and “southern lights,” and is caused by energetic particles from the sun trapped in Saturn’s magnetic field. 3 4 EXECUTIVE SUMMARY associates authored or co-authored a total of 204 refereed, scholarly papers in the disciplines’ most The Department of Astronomy teaches science to prestigious journals. hundreds of non-science majors from throughout the university, and runs one of the largest astronomy degree The funding of the Astronomy Department, the Center programs in the country. Research within the for Space Physics, and the Institute for Astrophysical Astronomy Department is thriving, and we retain our Research was changed this past year. In previous years, strong commitment to teaching and service. only the research centers received research funding, but last year the Department received a portion of this The Department graduated a class of twelve research funding based on grant activity by its faculty. -
Space Weather — History and Current Status
Space Weather — History and Current Status Ji Wu National Space Science Center, CAS Oct. 3, 2017 1 Contents 1. Beginning of Space Age and Dangerous Environment 2. The Dynamic Space Environment so far We Know 3. The Space Weather Concept and Current Programs 4. Looking at the Future Space Weather Programs 2 1.Beginning of Space Age and Dangerous Environment 3 Space Age Kai'erdishi Korolev Oct. 4, 1957, humanity‘s first artificial satellite, Sputnik-1, has launched, ushering in the Space Age. 4 Space Age Explorer 1 was the first satellite of the United States, launched on Jan 31, 1958, with scientific object to explore the radiation environment of geospace. 5 Unknown Space Environment Sputnik-2 (Nov 3, 1957) detected the Earth's outer radiation belt in the far northern latitudes, but researchers did not immediately realize the significance of the elevated radiation because Sputnik 2 passed through the Van Allen belt too far out of range of the Soviet tracking stations. Explorer-1 detected fewer cosmic rays in its orbit (which ranged from 220 miles from Earth to 1,563 miles) than Van Allen expected. 6 Space Age - unknown and dangerous space environment 7 Satellite failures due to the unknown and Particle dangerous space environment Radiation! Statistics show that the space radiation environment is one of the main causes of satellite failure. The space radiation environment caused about 2,300 satellite failures of all the 5000 failure events during the 1966-1994 period collected by the National Geophysical Data Center. Statistics of the United States in 1996 indicate that the space environment caused more than 40% of satellite failures in 1958-1986, and 36% in 1986-1996. -
Space Weather ± Physics and Effects Volker Bothmer and Ioannis A
Space Weather ± Physics and Effects Volker Bothmer and Ioannis A. Daglis Space Weather ± Physics and Effects Published in association with Praxis Publishing Chichester, UK Dr Volker Bothmer Dr Ioannis A. Daglis Institute for Astrophysics National Observatory of Athens University of GoÈttingen Athens GoÈ ttingen Greece Germany SPRINGER±PRAXIS BOOKS IN ENVIRONMENTAL SCIENCES SUBJECT ADVISORY EDITOR: John Mason B.Sc., M.Sc., Ph.D. ISBN 10: 3-540-23907-3 Springer-Verlag Berlin Heidelberg New York ISBN 13: 978-3-540-23907-9 Springer-Verlag Berlin Heidelberg New York Springer is part of Springer-Science + Business Media (springer.com) Bibliographic information published by Die Deutsche Bibliothek Die Deutsche Bibliothek lists this publication in the Deutsche Nationalbibliogra®e; detailed bibliographic data are available from the Internet at http://dnb.ddb.de Library of Congress Control Number: 2006921904 Apart from any fair dealing for the purposes of research or private study, or criticism or review, as permitted under the Copyright, Designs and Patents Act 1988, this publication may only be reproduced, stored or transmitted, in any form or by any means, with the prior permission in writing of the publishers, or in the case of reprographic reproduction in accordance with the terms of licences issued by the Copyright Licensing Agency. Enquiries concerning reproduction outside those terms should be sent to the publishers. # Praxis Publishing Ltd, Chichester, UK, 2007 Printed in Germany The use of general descriptive names, registered names, trademarks, etc. in this publication does not imply, even in the absence of a speci®c statement, that such names are exempt from the relevant protective laws and regulations and therefore free for general use. -
NASA Living with a Star TR&T Town Hall Renaissance Washington, DC
NASA Living With a Star TR&T Town Hall Renaissance Washington, DC Downtown 13 December, 2018 LWS Town Hall Agenda Item Time Agenda Item Presenter 1 6:30 PM Welcome Nicky Fox 2 6:35 PM LPAG Status Anthea Coster / Mark Linton 3 6:55 PM LWS Program Status Jeff Morrill / Simon Plunkett 4 7:15 PM Multi-Agency Collaborations & Space Janet Kozyra Weather Activities 5 7:30 PM GDC STDT Status Jared Leisner 6 7:45 PM LWS Infrastructure & New Initiatives Lika Guhathakurtha 7 8:00 PM ADJOURN 2 LWS Town Hall Agenda Item Time Agenda Item Presenter 1 6:30 PM Welcome Nicky Fox 2 6:35 PM LPAG Status Anthea Coster / Mark Linton 3 6:55 PM LWS Program Status Jeff Morrill / Simon Plunkett 4 7:15 PM Multi-Agency Collaborations & Space Janet Kozyra Weather Activities 5 7:30 PM GDC STDT Status Jared Leisner 6 7:45 PM LWS Infrastructure & New Initiatives Lika Guhathakurtha 7 8:00 PM ADJOURN 3 NASA Living with a Star Program Analysis Group (LPAG) Executive Committee (EC) Co-Chairs: • Anthea Coster (MIT Haystack Observatory) • Mark Linton (Naval Research Laboratory, NRL) EC Members: • Joe Borovsky (Space Science Institute) • Richard Collins (University of Alaska) • Seebany Datta-Barua (Illinois Institute of Technology) • Matina Gkioulidou (JHU / APL) • Fan Guo (Los Alamos National Laboratory) • Jorg-Micha Jahn (Southwest Research Institute) • Enrico Landi (University of Michigan) • John Leibacher (National Solar Observatory) • Sabrina Savage (NASA / MSFC) • Brian Walsh (Boston University) See website at https://lwstrt.gsfc.nasa.gov/lpag 4 Living with a Star LPAG, continued Liaison Members: Charles N. -
NASA's Office of Space Science and Applications: Process, Priorities and Goals
NASA's Office of Space Science and Applications: Process, Priorities and Goals January 1992 NTIS order #PB92-152503 Office of Technology Assessment Congressional Board of the 102d Congress GEORGE E. BROWN, JR., California, Chairman TED STEVENS, Alaska, Vice Chairman Senate House EDWARD M. KENNEDY JOHN 0. DINGELL Massachusetts Michigan ERNEST F. HOLLINGS CLARENCE E. MILLER South Carolina Ohio CLAIBORNE PELL DON SUNDQUIST Rhode Island Tennessee ORRIN G. HATCH AMO HOUGHTON Utah New York CHARLES E. GRASSLEY (Vacancy) Iowa JOHN H. GIBB0NS (Nonvoting) Advisory Council CHASE N. PETERSON, Chairman MICHEL T. HALBOUTY MAX LENNON University of Utah Chairman of the Board & President Salt Lake City, Utah Chief Executive Officer Clemson University Michel T Halbouty Energy Co. Clemson, South Carolina Houston, Texas JOSHUA LEDERBERG, Vice Chairman - NEIL E. HARL JOSEPH E. ROSS Professor Professor Director Rockefeller University Department of Economics Congressional Research Service New York, New York Iowa State University The Library of Congress Ames,. Iowa Washington, D.C. CHARLES A. BOWSHER JAMES C. HUNT JOHN F.M. SIMS Comptroller General of Chancellor Vice President Marketing the United States Health Sciences Center Usibelli Coal Mine, Inc. Washington, D.C. University of Tennessee Fairbanks, Alaska Memphis, Tennessee LEWIS M. BRANSCOMB HENRY KOFFLER MARINA v.N. WHITMAN Director of Science, Technology & President Emeritus Vice President & Group Executive Public Policy Program University of Arizona Public Affairs Staffs Group Albert Pratt Public Service -
The Hubble Space Telescope Aurora C
Instrumentation and Facilities Research Students have access to rooftop instructional optical and radio telescopes in the Judson B. The Department of Astronomy, through its affiliated research units (the CSP, the IAR, and CISM), has a robust and thriving research program. Research areas in the Department include observational and Coit Observatory. Through the B.U.-Lowell Observatory partnership we have guaranteed time theoretical studies in a variety of fields. To learn about the research programs of individual faculty-led groups, go to www.bu.edu/astronomy/research. A few of the current research projects at BU are: on the 1.8m Perkins telescope and the 4.3m DCT near Flagstaff, AZ. We are frequently guest investigators at national and international facilities in Puerto Rico, Chile, Hawaii, Massachusetts, The Hubble Space Telescope Arizona, New Mexico, West Virginia, and Australia. Members of the Department also use Aurora Campaign space-based facilities, such as NASA’s Hubble Space Telescope; the Fermi Gamma-ray Space The aurora on Jupiter and Saturn Telescope; the Cluster suite of four satellites; the Chandra X-Ray Observatory; and Spitzer, have been studied with increasing Herschel, and Swift satellites. sensitivity and resolution in a series of HST Guest Observer programs of UV imaging and spectroscopy. These have revolutionized our understanding of the auroral phenomenona on both planets, and the auroral emissions provide the only remote method to study the magnetospheres of these giant planets. Discovery Channel Telescope (DCT) Boston University has recently partnered with Lowell Observatory in the Discovery Channel Telescope (DCT). The BU Astronomy Department will have guaranteed observing time on the 4.3 meter telescope, located on a site called “Happy Jack” about 40 miles from Flagstaff, AZ. -
The Dalton Minimum and John Dalton's Auroral Observations
Silverman and Hayakawa (2021) The Dalton Minimum and John Dalton’s Auroral Observations, Journal of Space Weather and Space Climate. DOI: 10.1051/swsc/2020082 The Dalton Minimum and John Dalton’s Auroral Observations Sam M. Silverman 18 Ingleside Road Lexington, MA [email protected] Hisashi Hayakawa (1) Institute for Space-Earth Environmental Research Nagoya University, Nagoya, 4648601, Japan (2) Institute for Advanced Researches Nagoya University, Nagoya, 4648601, Japan (3) UK Solar System Data Centre, Space Physics and Operations Division, RAL Space, Science and Technology Facilities Council, Rutherford Appleton Laboratory, Harwell Oxford, Didcot, Oxfordshire, OX11 0QX, UK [email protected] 1 Silverman and Hayakawa (2021) The Dalton Minimum and John Dalton’s Auroral Observations, Journal of Space Weather and Space Climate. DOI: 10.1051/swsc/2020082 Abstract In addition to the regular Schwabe cycles of approximately 11 y, “prolonged solar activity minima” have been identified through the direct observation of sunspots and aurorae, as well as proxy data of cosmogenic isotopes. Some of these minima have been regarded as grand solar minima, which are arguably associated with the special state of the solar dynamo and have attracted significant scientific interest. In this paper, we review how these prolonged solar activity minima have been identified. In particular, we focus on the Dalton Minimum, which is named after John Dalton. We review Dalton’s scientific achievements, particularly in geophysics. Special emphasis is placed on his lifelong observations of auroral displays over approximately five decades in Great Britain. Dalton’s observations for the auroral frequency allowed him to notice the scarcity of auroral displays in the early 19th century. -
On-Line Education Material at CCMC
On-Line Education Material at CCMC George Siscoe, M. Kuznetsova, A. Chulaki, D. Berrios A. Taktakishvili, A. Pulkkinen, L. Rastaetter, M. Hesse M. Maddox, P. MacNeice Outline • CCMC Support of Heliophysics Summer School • General Purpose Runs for Education and Research • Idea of On-line Educational Courses on Space Physics and Space Weather that Use CCMC Simulations • DEMO of the First of the Series of Modules on Earth’s Magnetosphere (George Siscoe, Anna Chulaki) George Siscoe George Siscoe Sandro Taktakishvili Antti Pulkkinen SOHO Satellite Instrument Images for 4 Moments of time t1<t2<t3<t4. EIT‐ EUV Imaging Telescope LASCO C2 Coronograph LASCO C3 Coronograph t1 t2 t3 t4 5 ExerciseExercise 11:: WhichWhich instrumentinstrument hashas dedetectedtected ththee CMECME appearanceappearance firstfirst andand whwhen?en? Average CME Speed from the Catalog at IACS CUA (http://cdaw.gsfc.nasa.gov/CME_list/) (Exercise 4) There were 8 halo CMEs in March and April of 2002 listed in this catalog. CME date Catalog POS speed TheThe tabltablee showsshows halohalo CMECMEss inin MarchMarch andand AprilApril 20022002 listedlisted At 2 Rs inin ththee catalog,catalog, justjust bebeforefore 2002/04/172002/04/17 CMECME occurred.occurred. (km/sec) OneOne ofof thethe waysways ofof forecastingforecasting CMECME arrivalarrival timestimes isis toto 2002/03/10 1429 estimateestimate ththee propagationpropagation timtimee ofof anan “average”“average”sspeedpeed 2002/03/11 950 CME.CME. 2002/03/14 907 ExerciseExercise 4:4: TakingTaking thethe averageaverage ofof halohalo CMECME speedsspeeds listedlisted inin thethe TableTable 2002/03/15 957 estimateestimate thethe arrivalarrival timetime ofof thethe “average”“average” sspeedpeed CMECME toto thethe EarthEarth andand comparecompare itit toto thethe observedobserved arrivalarrival timetime andand thethe arrivalarrival timetime obtainedobtained inin thethe ExercisExercisee 3.3. -
The Boston University Astronomy Department Annual Report 2006
The Boston University Astronomy Department Annual Report 2006 Dept. Administrator: Susanna Lamey Chairman: James M. Jackson The Boston University Astronomy Department 2006 Annual Report - July 2005-June 2006 TABLE OF CONTENTS Executive Summary 4 Faculty and Staff 5 Teaching 6 Observatory and Facilities 9 Boston University Astronomical Society 10 The Graduate Program 11 Seminar Series 12 Alumni Affairs and Public Outreach 12 Research 13 Future Plans and Departmental Needs 14 APPENDIX A: Faculty, Staff, and Graduate Students 19 APPENDIX B: 2006 Astronomy Graduates 21 APPENDIX C: Seminar Series 2005/2006 22 APPENDIX D: Sponsored Project Funding 24 APPENDIX E: PhD Recipients, 1995-2006 26 APPENDIX F: Research Telescopes Available to Peer Institutions 30 The Boston University Astronomy Department 2006 Annual Report - July 2005-June 2006 EXECUTIVE SUMMARY The Department of Astronomy teaches science to hundreds of non-science majors from throughout the university, and runs one of the largest astronomy degree programs in the country. The faculty’s substantial grant activity, scientific productivity, and professional ser- vice demonstrate the Department’s excellence. The Department graduated a class of nine undergraduates, all with a major concentration, as well as two Astronomy minors. While our program is small by CAS standards, among astronomy programs it is among the largest in the US. In our graduate program, two students completed a PhD. Our graduate recruiting for next year was very successful: we have an incoming class of ten new students, bringing our total of new and ongoing astronomy graduate students to . Overall grant revenue won through competitive proposals by Department Faculty and affili- ated Research Associates stayed at modern high levels. -
Comparative Magnetospheres: Lessons for Earth
Advances in Space Research 33 (2004) 2113–2120 www.elsevier.com/locate/asr Comparative magnetospheres: lessons for Earth V.M. Vasyliunas * Max-Planck-lnstitut fu€r Aeronomie, Max-Planck-Str. 2, 37191 Katlenburg-Lindau, Germany Received 3 March 2003; received in revised form 3 April 2003; accepted 3 April 2003 Abstract The comparative study of various magnetospheres is not only interesting in itself but also useful for understanding better many aspects of the magnetosphere of Earth. Scaling relations can be tested over a much wider parameter range than that provided by Earth alone. Comparison of Earth with other magnetospheres tells us about the relative importance of the ionosphere, the sig- nificance of kinetic effects, the dependence on the geometrical configuration of rotation axis, magnetic dipole, and solar wind flow direction, and the role of the external magnetic field. Processes such as magnetic field line reconnection and auroral particle ac- celeration are common to many different magneto-spheres and can be better understood when observed in different contexts. Diffusive transport across closed magnetic field lines plays a special role in the magnetospheres both of Jupiter and of Earth. Finally, some properties and limitations of numerical simulation work are illuminated by application under distinct conditions provided by different magnetospheres, in particular Jupiter and Earth. Ó 2004 COSPAR. Published by Elsevier Ltd. All rights reserved. Keywords: Comparative magnetospheres; Lessons for Earth; Scaling relations; Planetary magnetospheres 1. Introduction significance; the first step in trying to understand a magnetosphere that is being observed for the first time Lessons for Earth, or lessons from Earth? Both are has always been to invoke analogies from the terrestrial objectives of comparative magnetosphere studies as magnetosphere and see how far they can be pushed. -
Dr. Gary M. Erickson
Dr. Gary M. Erickson Biography: Gary Erickson received his B.S. (1976) and M.S. (1978) degrees in physics from Florida Atlantic University, and his Ph.D. (1985) in space physics from Rice University. After a post-doctoral ap- pointment at Rice University (1985{1986), he was an NRC Resident Research Associate at Goddard Space Flight Center (1986{1987), a Geophysics Scholar at the Air Force Geophysical Laboratory (1987{1989), and Senior Scientist at Massachusetts Technological Laboratory (1989{1990). He came to Boston University as a Research Associate (1990{1991), taught a graduate course in mag- netospheric physics, and became a member of the Research Faculty in the Department of Space Physics and Astronomy and Center for Space Physics (1991{2003). He held Visiting Faculty ap- pointments at the Air Force Research Laboratory under the Air Force Summer Faculty Research Program (1997, 2001, 2002). He is now a Research Scientist at the Solar Observatory and Visiting Associate Professor of Physics at Prairie View A&M University (PVAMU). Research has included both theoretical and data-analysis projects. Theoretical projects include convection in Earth's plasma sheet, the physics of the Harang discontinuity, the generation of magnetic-¯eld-aligned currents, magnetospheric structure, and magnetopause reconnection and turbulence. The problem of the magnetospheric substorm has been a particular research focus since he elucidated the \pressure-balance inconsistency" as a graduate student. For his Ph.D. project, he developed the ¯rst computer code to model force-balanced, adiabatic, magnetospheric convection, which established the structure of the substorm growth phase. Data-analysis projects include use of data from the CRRES and Geotail satellites to describe magnetospheric substorms. -
The Heliophysics Summer School: 10 Years and Counting
The Heliophysics Summer School: 10 years and Counting By Dr. Tony Phillips Some institutions of cutting-edge learning are very old. Harvard: 380 years. Princeton: 270 years. Caltech: 125 years. Others are a little younger. This year, academicians around the world are celebrating the 10th anniversary of the “Heliophysics Summer School,” a fresh-faced academy that introduces the next generation of scientists to a field of study that, arguably, didn’t even exist when the new millennium began. “Heliophysics is something new and exciting,” says Lika Guhathakurta of NASA Headquarters. “It’s a leap across scientific boundaries,” says Karel Schrijver, formerly of the Lockheed Martin Solar & Astrophysics Laboratory. “It is a blueprint for the Universe,” says Amitava Bhattacharjee, Professor of Astrophysical Sciences at Princeton University. It begins with Helios, our sun. Of all the objects in the cosmos, the sun affects our planet most. It is the 900lb gorilla of the Solar System, shaping climate, weather, even life itself. Earth and the sun are deeply and intricately connected, not only by simple rays of light and heat, but also by a complex web of electricity, magnetism, solar wind and extreme ultraviolet radiation. Lines of electrical current and magnetic force can sometimes be traced, without interruption, all the way from the ground beneath our feet to the base of seething sunspots 93 million miles away. Our planet and our star are, in a sense, one. “Back in the early 2000s, NASA had a division called the ‘Sun-Earth connection,’ which recognized this link,” recalls Guhathakurta. ”When Mike Griffin became the NASA administrator in April of 2005, he asked us to come up with a one-word description of our division that captured both the holistic simplicity and the vast scope of the sun-Earth system.