And Long-Wavelength-Sensitive Cones Derived from Measurements in Observers of Known Genotype
Total Page:16
File Type:pdf, Size:1020Kb
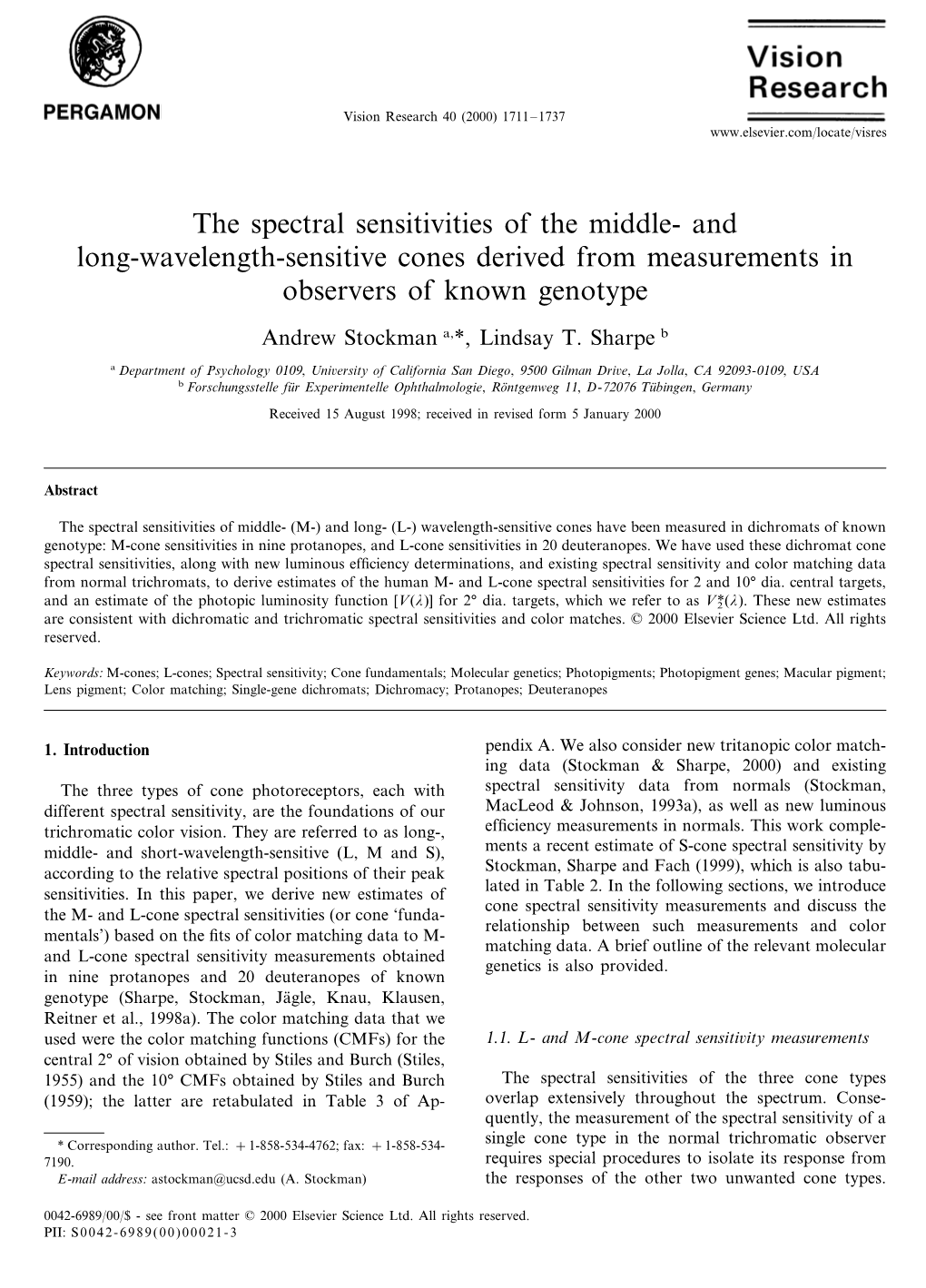
Load more
Recommended publications
-
Chromatic Function of the Cones D H Foster, University of Manchester, Manchester, UK
Chromatic Function of the Cones D H Foster, University of Manchester, Manchester, UK ã 2010 Elsevier Ltd. All rights reserved. Glossary length. If l is the path length and a(l) is the spectral absorptivity, then, for a homogeneous isotropic CIE, Commission Internationale de l’Eclairage – absorbing medium, A(l)=la(l) (Lambert’s law). The CIE is an independent, nonprofit organization Spectral absorptance a(l) – Ratio of the spectral responsible for the international coordination of radiant flux absorbed by a layer to the spectral lighting-related technical standards, including radiant flux entering the layer. If t(l) is the spectral colorimetry standards. transmittance, then a(l)=1Àt(l). The value of a(l) Color-matching functions – Functions of depends on the length or thickness of the layer. For a wavelength l that describe the amounts of three homogeneous isotropic absorbing medium, fixed primary lights which, when mixed, match a a(l)=1Àt(l)=1À10Àla(l), where l is the path length monochromatic light of wavelength l of constant and a(l) is the spectral absorptivity. Changes in the radiant power. The amounts may be negative. The concentration of a photopigment have the same color-matching functions obtained with any two effect as changes in path length. different sets of primaries are related by a linear Spectral absorptivity a(l) – Spectral absorbance of transformation. Particular sets of color-matching a layer of unit thickness. Absorptivity is a functions have been standardized by the CIE. characteristic of the medium, that is, the Fundamental spectral sensitivities – The color- photopigment. Its numerical value depends on the matching functions corresponding to the spectral unit of length. -
Color Vision Mechanisms
11 COLOR VISION MECHANISMS Andrew Stockman Department of Visual Neuroscience UCL Institute of Opthalmology London, United KIngdom David H. Brainard Department of Psychology University of Pennsylvania Philadelphia, Pennsylvania 11.1 GLOSSARY Achromatic mechanism. Hypothetical psychophysical mechanisms, sometimes equated with the luminance mechanism, which respond primarily to changes in intensity. Note that achromatic mech- anisms may have spectrally opponent inputs, in addition to their primary nonopponent inputs. Bezold-Brücke hue shift. The shift in the hue of a stimulus toward either the yellow or blue invariant hues with increasing intensity. Bipolar mechanism. A mechanism, the response of which has two mutually exclusive types of out- put that depend on the balance between its two opposing inputs. Its response is nulled when its two inputs are balanced. Brightness. A perceptual measure of the apparent intensity of lights. Distinct from luminance in the sense that lights that appear equally bright are not necessarily of equal luminance. Cardinal directions. Stimulus directions in a three-dimensional color space that silence two of the three “cardinal mechanisms.” These are the isolating directions for the L+M, L–M, and S–(L+M) mech- anisms. Note that the isolating directions do not necessarily correspond to mechanism directions. Cardinal mechanisms. The second-site bipolar L–M and S–(L+M) chromatic mechanisms and the L+M luminance mechanism. Chromatic discrimination. Discrimination of a chromatic target from another target or back- ground, typically measured at equiluminance. Chromatic mechanism. Hypothetical psychophysical mechanisms that respond to chromatic stimuli, that is, to stimuli modulated at equiluminance. Color appearance. Subjective appearance of the hue, brightness, and saturation of objects or lights. -
Cone Fundamentals and CIE Standards
Cone fundamentals and CIE standards Andrew Stockman UCL Institute of Ophthalmology, London, UK Address UCL Institute of Ophthalmology, 11-43 Bath Street, London EC1V 9EL, UK Corresponding author: Stockman, Andrew ([email protected]) Abstract A knowledge of the spectral sensitivities of the long- (L-), middle- (M-) and short- (S-) wavelength-sensitive cone types is vital for modelling human color vision and for the practical applications of color matching and color specification. After being agnostic about defining standard cone spectral sensitivities, the Commission Internationale de l' Éclairage (CIE) has sanctioned the cone spectral sensitivity estimates of Stockman & Sharpe [1] and the associated measures of luminous efficiency [2,3] as “physiologically-relevant” standards for color vision [4,5]. These can be used to model mean normal color vision at the photoreceptor level and postreceptorally. Both LMS and XYZ versions have been defined for 2-deg and 10-deg vision. Built into the standards are corrections for individual differences in macular and lens pigment densities, but individual differences in photopigment optical density and the spectral position of the cone photopigments can also be accommodated [6,7]. Understanding the CIE standard and its advantages is of current interest and importance. 1 Color perception and photopic visual function are inextricably linked to and, indeed, limited by the properties of the three cone photoceptors: the long- (L-), middle- (M-) and short- (S-) wavelength-sensitive cones. This short review covers the derivation of the recent “physiologically- relevant” Commission Internationale de l' Éclairage (CIE) 2006; 2015 cone spectral sensitivities and luminous efficiency functions for 2-deg and 10-deg vision [4,5] and provides background details about cone spectral sensitivities and trichromatic color matching. -
Color Vision and Night Vision Chapter Dingcai Cao 10
Retinal Diagnostics Section 2 For additional online content visit http://www.expertconsult.com Color Vision and Night Vision Chapter Dingcai Cao 10 OVERVIEW ROD AND CONE FUNCTIONS Day vision and night vision are two separate modes of visual Differences in the anatomy and physiology (see Chapters 4, perception and the visual system shifts from one mode to the Autofluorescence imaging, and 9, Diagnostic ophthalmic ultra- other based on ambient light levels. Each mode is primarily sound) of the rod and cone systems underlie different visual mediated by one of two photoreceptor classes in the retina, i.e., functions and modes of visual perception. The rod photorecep- cones and rods. In day vision, visual perception is primarily tors are responsible for our exquisite sensitivity to light, operat- cone-mediated and perceptions are chromatic. In other words, ing over a 108 (100 millionfold) range of illumination from near color vision is present in the light levels of daytime. In night total darkness to daylight. Cones operate over a 1011 range of vision, visual perception is rod-mediated and perceptions are illumination, from moonlit night light levels to light levels that principally achromatic. Under dim illuminations, there is no are so high they bleach virtually all photopigments in the cones. obvious color vision and visual perceptions are graded varia- Together the rods and cones function over a 1014 range of illu- tions of light and dark. Historically, color vision has been studied mination. Depending on the relative activity of rods and cones, as the salient feature of day vision and there has been emphasis a light level can be characterized as photopic (cones alone on analysis of cone activities in color vision. -
Radiometry and Photometry
Radiometry and Photometry Wei-Chih Wang Department of Power Mechanical Engineering National TsingHua University W. Wang Materials Covered • Radiometry - Radiant Flux - Radiant Intensity - Irradiance - Radiance • Photometry - luminous Flux - luminous Intensity - Illuminance - luminance Conversion from radiometric and photometric W. Wang Radiometry Radiometry is the detection and measurement of light waves in the optical portion of the electromagnetic spectrum which is further divided into ultraviolet, visible, and infrared light. Example of a typical radiometer 3 W. Wang Photometry All light measurement is considered radiometry with photometry being a special subset of radiometry weighted for a typical human eye response. Example of a typical photometer 4 W. Wang Human Eyes Figure shows a schematic illustration of the human eye (Encyclopedia Britannica, 1994). The inside of the eyeball is clad by the retina, which is the light-sensitive part of the eye. The illustration also shows the fovea, a cone-rich central region of the retina which affords the high acuteness of central vision. Figure also shows the cell structure of the retina including the light-sensitive rod cells and cone cells. Also shown are the ganglion cells and nerve fibers that transmit the visual information to the brain. Rod cells are more abundant and more light sensitive than cone cells. Rods are 5 sensitive over the entire visible spectrum. W. Wang There are three types of cone cells, namely cone cells sensitive in the red, green, and blue spectral range. The approximate spectral sensitivity functions of the rods and three types or cones are shown in the figure above 6 W. Wang Eye sensitivity function The conversion between radiometric and photometric units is provided by the luminous efficiency function or eye sensitivity function, V(λ). -
The Eye and Night Vision
Source: http://www.aoa.org/x5352.xml Print This Page The Eye and Night Vision (This article has been adapted from the excellent USAF Special Report, AL-SR-1992-0002, "Night Vision Manual for the Flight Surgeon", written by Robert E. Miller II, Col, USAF, (RET) and Thomas J. Tredici, Col, USAF, (RET)) THE EYE The basic structure of the eye is shown in Figure 1. The anterior portion of the eye is essentially a lens system, made up of the cornea and crystalline lens, whose primary purpose is to focus light onto the retina. The retina contains receptor cells, rods and cones, which, when stimulated by light, send signals to the brain. These signals are subsequently interpreted as vision. Most of the receptors are rods, which are found predominately in the periphery of the retina, whereas the cones are located mostly in the center and near periphery of the retina. Although there are approximately 17 rods for every cone, the cones, concentrated centrally, allow resolution of fine detail and color discrimination. The rods cannot distinguish colors and have poor resolution, but they have a much higher sensitivity to light than the cones. DAY VERSUS NIGHT VISION According to a widely held theory of vision, the rods are responsible for vision under very dim levels of illumination (scotopic vision) and the cones function at higher illumination levels (photopic vision). Photopic vision provides the capability for seeing color and resolving fine detail (20/20 of better), but it functions only in good illumination. Scotopic vision is of poorer quality; it is limited by reduced resolution ( 20/200 or less) and provides the ability to discriminate only between shades of black and white. -
Application Note
How Light Meters Can Fool Us A primer on why bluer white light looks brighter to us and how it can help save the planet Imagine two identical rooms artificially illuminated with exactly the same amount of white light. A light meter confirms that the two rooms have equal light levels. In fact, everything about the rooms is the same except for one important detail: The light in one room has a little more blue in it than the other room. Why, then, does the room with the bluer light seem so much brighter? (See Fig. 1.) For decades this effect has created more than a little controversy among vision scientists, with many dismissing it as nothing more than an illusion. But recent advances in vision science now support the view that this is a real effect. The room with the bluer light does appear brighter to the human eye — a fact that can help reduce lighting costs by as much as 40% or more. To understand why, we need to know a little more about the history of why light meters are calibrated the way they are and how our eyes react to light. Figure 1: The shopping center above was initially lighted by fluorescent lamps having a color temperature of 4100K (left photo). The fluorescents were replaced by LEDs with a color temperature of 6000K (right photo). Even though the LED lamps use less electricity, the area still looks brighter because LED lighting is more efficient, but also because the higher color temperature creates a bluer white light that looks brighter to us. -
Photoreceptor Spectral Sensitivities: Common Shape in the Long-Wavelength Region T
Vision Res. Vol. 35, No. 22, pp. 3083-3091, 1995 ~ Pergamon Copyright © 1995Elsevier Science Ltd 0042-6989(95)00114-X Printed in Great Britain. All rights reserved 0042-6989/95 $9.50+ 0.00 Photoreceptor Spectral Sensitivities: Common Shape in the Long-wavelength Region T. D. LAMB* Received 16 January 1995; in revised form 24 March 1995 Previous measurements of mammalian photoreceptor spectral sensitivity have been analysed, with particular attention to the long-wavelength region. The measurements selected for study come from rod and cone systems, and from human, monkey, bovine and squirrel sources. For the spectra from photoreceptor electrophysiology and from psychophysical sensitivity, the frequency sealing applied by Mansfield (1985, The visual system, pp. 89-106. New York: Alan Liss) provides a common shape over a range of at least 7 log10 units of sensitivity, from low frequencies (long wavelengths) to frequencies beyond the peak. The same curve is applicable to the absorbance spectrum of bovine rhodopsin, although the ahsorbance can only be measured down to about 2 lOglo units below the peak. At the longest wavelengths the results exhibit a common limiting slope of 70 log, units (or 30.4 log~0 units) per unit of normalized frequency. A simple equation is presented as a generic description for the ~-band of mammalian photoreceptor spectral sensitivity curves, and it seem likely that the equation may be equally applicable to retinalrbased pigments in other species. Despite the lack of a theoretical basis, the equation has the correct asymptotic behaviour at long wavelengths, and it provides an accurate description of the peak. -
Night Vision in the Elderly: Consequences for Seeing Through a ‘‘Blue Filtering’’ Intraocular Lens
1518 PERSPECTIVE Br J Ophthalmol: first published as 10.1136/bjo.2005.073734 on 18 October 2005. Downloaded from Night vision in the elderly: consequences for seeing through a ‘‘blue filtering’’ intraocular lens J S Werner ............................................................................................................................... Br J Ophthalmol 2005;89:1518–1521. doi: 10.1136/bjo.2005.073734 Relative scotopic spectral sensitivity depends only on the spectral sensitivity maximum. Their analyses considered the percentage change in sensitivity. rhodopsin photopigment and ocular media absorption Here, we shall consider the same issue, but spectra. Rhodopsin is well characterised so the relative illustrated more traditionally in logarithmic units scotopic spectral sensitivity function can be calculated for so that the losses can be seen in the context of the full range of scotopic sensitivity. It will be intraocular lenses (IOLs) of known spectral density. In a shown why IOL absorption of light at wave- recent perspective, Mainster and Sparrow concluded that lengths near the sensitivity maximum is no more an IOL with short wave absorbing chromophores would important for scotopic vision than absorption at any other wavelengths. We shall also consider provide more retinal protection than conventional IOLs, but some aspects of scotopic vision not discussed by the practical consequences for scotopic vision are unclear. Mainster and Sparrow. In particular, the con- This paper uses published experiments to examine the sequences of IOL absorption spectra are evalu- ated in terms of scotopic spatial contrast implications for scotopic vision of the IOLs analysed by sensitivity as a quantitative index of pattern, or Mainster and Sparrow. A 14.6% reduction in scotopic form, vision. sensitivity is expected for a SN60AT (AcrySof Natural) compared to a SA60AT (Conventional AcrySof) IOL under SCOTOPIC SPECTRAL SENSITIVITY WITH AN IOL CONTAINING CHROMOPHORES broadband illumination (equal quantum spectrum). -
The Influence of Different Photometric Observers on Luxmeter Accuracy for Leds and Fls Lamps Measurements
Optica Applicata, Vol. XLIX, No. 2, 2019 DOI: 10.5277/oa190214 The influence of different photometric observers on luxmeter accuracy for LEDs and FLs lamps measurements 1* 2 IRENA FRYC , PRZEMYSLAW TABAKA 1Electrical Engineering Faculty, Bialystok University of Technology, Wiejska 45d, 15-351 Bialystok, Poland 2Institute of Electrical Power Engineering, Lodz University of Technology, Stefanowskiego 18/22, 90-924 Lodz, Poland *Corresponding author: [email protected] Age-dependent changes in human eye spectral sensitivity play an important role in contemporary lighting research. Nowadays lighting designing practices take into account the fact that an illumi- nance level perceived by a given observer depends also on his age. According to recommendations of the International Commission on Illumination CIE presented in the document 227:2017, users age should be taken into account in lighting design of public buildings. The natural consequence of this approach in designing should be the fact that the age of a user also should be considered when verification of lighting installation photometric parameters is performed. According to rec- ommendations of latest CIE documents, verifications of illuminance levels are performed by lux- meters whose spectral sensitivity matches the CIE standard photometric observer V(λ) function. At present there is a lack of papers describing how the accuracy of illuminance measurements could be affected by the fact that with age there are changes in human eye spectral sensitivity and it differs from V(λ) function. To fill this gap we present in this article results showing how applying of dif- ferent photometric observers influence the luxmeter accuracy. The calculations were performed for LED and FL light sources measured by class B and class C luxmeters. -
Vision and Exterior Lighting: Shining Some Light on Scotopic and Photopic Lumens in Roadway Conditions
Vision and Exterior Lighting: Shining Some Light on Scotopic and Photopic Lumens in Roadway Conditions Dr. Jack Josefowicz and Ms. Debbie Ha November 2008 This white paper, authored by Dr. Jack Josefowicz and Ms. Debbie Ha, has been reviewed by Dr. Samuel M. Berman and he concurs that the technical and scientific information therein is consistent with generally accepted knowledge in lighting and vision science. Summary The human eye contains 2 major light sensitive photoreceptors, namely cones and rods each with its own spectral sensitivity, photopic sensitivity for cones and scotopic sensitivity for rods. At light levels typical of night time roadway lighting, both cones and rods can be active and both spectral sensitivities could apply. However for straight ahead viewing where the line of sight is directed to distant object detection and recognition, such as a pedestrian on a roadway, only cones are relevant. In that case, the photopic function is the operating sensitivity. The rod response, along with scotopic sensitivity, does not contribute to the important visual task of direct object recognition. Other visual tasks such as large area brightness perception, peripheral guidance and detection of objects not in the line of sight are affected by rod response and in that case both photopic and scotopic sensitivity functions need to be included to correctly characterize how light affects vision. Overview Objects are best seen and discerned in central vision i.e. when we directly view them straight ahead. In night time conditions, this primary visual task will generally benefit from the presence of an exterior lighting system. However, when we look straight ahead, the most important light coming from the viewed object activates the very center of the retina known as the fovea. -
The Spectral Sensitivity of Dark- and Light-Adapted Cat Retinal Ganglion Cells
The Journal of Neuroscience, April 1993, 73(4): 1543-i 550 The Spectral Sensitivity of Dark- and Light-adapted Cat Retinal Ganglion Cells Elke Guenther and Eberhart Zrenner Department of Pathophysiology of Vision and Neuroophthalmology, Division of Experimental Ophthalmology, University Eye Hospital, 7400 Ttibingen, Germany The spectral sensitivity of cat retinal ganglion neurons (RGNs) red and green lights (Sechzer and Brown, 1964; Meyer and An- was determined by means of extracellular recordings under derson, 1965) and also between red and blue or yellow lights scotopic and photopic conditions, in both receptive field (Mellow and Peterson, 1964). center and surround. Test stimuli were presented either as Basedon the finding that rod and cone signalsconverge upon square-wave single flashes or as flicker stimuli. Chromatic retinal ganglioncells, asdemonstrated morphologically (Polyak, adaptation was achieved by a large steady monochromatic 1941; Walls, 1942; Rodieck, 1973) as well as physiologically background field. In the dark-adapted state the spectral sen- (Granit, 1943, 1947; Donner, 1950), various authors have sug- sitivity of the majority of ganglion cells (92%) was rod gested the cat’s ability of color discrimination in the mesopic mediated (peak sensitivity at 501 nm). Under photopic con- rangeto be mediated by an interaction between rods and long- ditions all neurons received input from a long-wavelength- wavelength cones (L-cones) only (Daw and Pearlman, 1969; sensitive (L-cone) system with a peak sensitivity of 550 nm. Andrews and Hammond, 1970a,b).However, someyears earlier Input from a short-wavelength-sensitive (S-cone) system Granit (1943) already had postulated in his dominator-modu- (peak sensitivity at 450 nm), however, was found only in 15% lator theory the existence of various cone systems.This idea of the ganglion cells.