Comparative Analysis Reveals Gravity Is Involved in the MIZ1-Regulated Root Hydrotropism
Total Page:16
File Type:pdf, Size:1020Kb
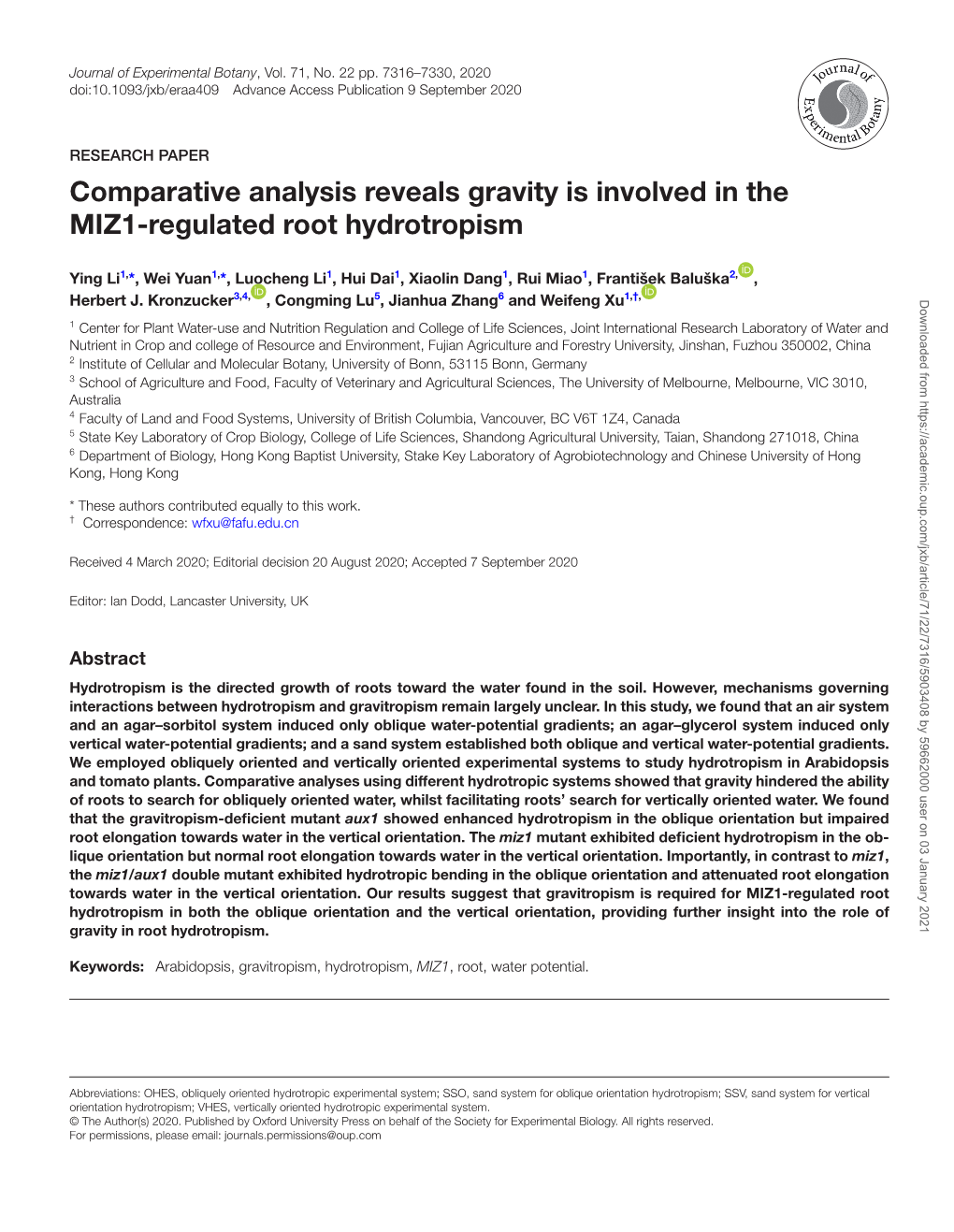
Load more
Recommended publications
-
Plant Tropisms: Providing the Power of Movement to a Sessile Organism
Int. J. Dev. Biol. 49: 665-674 (2005) doi: 10.1387/ijdb.052028ce Plant tropisms: providing the power of movement to a sessile organism C. ALEX ESMON, ULLAS V. PEDMALE and EMMANUEL LISCUM* University of Missouri-Columbia, Division of Biological Sciences, Columbia, Missouri, USA ABSTRACT In an attempt to compensate for their sessile nature, plants have developed growth responses to deal with the copious and rapid changes in their environment. These responses are known as tropisms and they are marked by a directional growth response that is the result of differential cellular growth and development in response to an external stimulation such as light, gravity or touch. While the mechanics of tropic growth and subsequent development have been the topic of debate for more than a hundred years, only recently have researchers been able to make strides in understanding how plants perceive and respond to tropic stimulations, thanks in large part to mutant analysis and recent advances in genomics. This paper focuses on the recent advances in four of the best-understood tropic responses and how each affects plant growth and develop- ment: phototropism, gravitropism, thigmotropism and hydrotropism. While progress has been made in deciphering the events between tropic stimulation signal perception and each character- istic growth response, there are many areas that remain unclear, some of which will be discussed herein. As has become evident, each tropic response pathway exhibits distinguishing characteris- tics. However, these pathways of tropic perception and response also have overlapping compo- nents – a fact that is certainly related to the necessity for pathway integration given the ever- changing environment that surrounds every plant. -
Carnivorous Plant Newsletter Vol 47 No 1 March 2018
The use of the right words: why carnitropism is inaccurate for carnivorous plants. Suggestion to reject the term “carnitropism” Aurélien Bour • Tropical botanic collections • Conservatoire et Jardins Botaniques de Nancy • 100 rue du Jardin Botanique • 54 600 Villers-lès-Nancy • France • [email protected] Keywords: carnitropism, chemonasty, chemotropism, plant movement, thigmonasty, tropism. Abstract: In the September 2017 issue of Carnivorous Plant Newsletter, the term ‘carnitropism’ was proposed to name triggered movements occurring in Dionaea, Drosera, and Pinguicula gen- era. However, those phenomena were misconceived and their interpretation lacked scientific back- ground, misleading to a flawed conclusion. Therefore, the present paper recommends to avoid the use of that term and recalls both the mechanisms behind plant movements and their associated names. Introduction Simón (2017) explained that “Plants are known to move towards certain stimuli (water, light, gravity) which are beneficial and these movements have been labeled hydrotropism, phototropism, geotropism.” Yet, such a statement is very likely to bring confusion. For one thing, no definition of tropism including its physiological origin was clearly introduced in the article. A couple of exam- ples cannot make up for that absence. For another, although tropism is indeed an instance of plant reaction to stimuli, that is not the sole one. Another kind of reaction, called “nastic movement” is actually more common when it comes to carnivorous plants. The main difference between these two mechanisms is found at the cell level, and could roughly be defined as such: • Tropism is usually a growth movement due to uneven cell multiplication, which eventually leads to the organ orientation. -
Root Tropisms: New Insights Leading the Growth Direction of the Hidden Half
plants Editorial Root Tropisms: New Insights Leading the Growth Direction of the Hidden Half Luigi Gennaro Izzo * and Giovanna Aronne Department of Agricultural Sciences, University of Naples Federico II, Via Università 100, 80055 Portici, Italy; [email protected] * Correspondence: [email protected] Abstract: Tropisms are essential responses of plants, orienting growth according to a wide range of stimuli. Recently, considerable attention has been paid to root tropisms, not only to improve cultivation systems, such as those developed for plant-based life support systems for future space programs, but also to increase the efficiency of root apparatus in water and nutrient uptake in crops on Earth. To date, the Cholodny–Went theory of differential auxin distribution remains the principal tropistic mechanism, but recent findings suggest that it is not generally applicable to all root tropisms, and new molecular pathways are under discussion. Therefore, an in-depth understanding of the mechanisms and functions underlying root tropisms is needed. Contributions to this special issue aimed to embrace reviews and research articles that deepen molecular, physiological, and anatom- ical processes orchestrating root tropisms from perception of the stimulus to bending. The new insights will help in elucidating plant–environment interactions, providing potential applications to improve plant growth on Earth and in space where microgravity diminishes or nullifies the gravitropism dominance. Keywords: directional growth; tropistic stimuli; root bending; Cholodny–Went; statoliths; auxin; altered gravity; microgravity Citation: Izzo, L.G.; Aronne, G. Root Tropisms: New Insights Leading the 1. Introduction Growth Direction of the Hidden Half. Plants 2021, 10, 220. https://doi.org/ Plant development is largely influenced by tropisms which govern organ position 10.3390/plants10020220 and growth during the whole life cycle. -
Hormonal Interactions During Root Tropic Growth: Hydrotropism Versus Gravitropism
Plant Mol Biol (2009) 69:489–502 DOI 10.1007/s11103-008-9438-x Hormonal interactions during root tropic growth: hydrotropism versus gravitropism Hideyuki Takahashi Æ Yutaka Miyazawa Æ Nobuharu Fujii Received: 30 October 2008 / Accepted: 17 November 2008 / Published online: 16 December 2008 Ó Springer Science+Business Media B.V. 2008 Abstract Terrestrial plants have evolved remarkable Introduction morphological plasticity that enables them to adapt to their surroundings. One of the most important traits that plants Survival of all living organisms depends on water avail- have acquired is the ability to sense environmental cues ability. To survive in terrestrial conditions, and given their and use them as a basis for governing their growth orien- sessile nature, plants establish a root system that obtains tation. The directional growth of plant organs relative to water from the soil. Plant roots display tropisms in the direction of environmental stimuli is a tropism. The response to the direction or gradient of environmental cues, Cholodny–Went theory proposes that auxin plays a key and these tropisms control the orientation of root growth. role in several tropisms. Recent molecular genetic studies Roots respond to gravity, light, water (moisture gradient), have strongly supported this hypothesis for gravitropism. touch (mechanical stimuli), temperature, electric fields, However, the molecular mechanisms of other tropisms are magnetic fields, and chemicals by gravitropism, phototro- far less clear. Hydrotropism is the response of roots to a pism, hydrotropism, thigmotropism, thermotropism, moisture gradient. Since its re-discovery in 1985, root electrotropism, magnetotropism and chemotropism, hydrotropism has been shown to be common among higher respectively (Hart 1990; edited in Gilroy and Masson plant species. -
Immediate Response to Stimulus
BIOLOGY CONTROL AND COORDINATION Chemical Coordination in Plants Plants do not have nervous system. They exhibit different mechanisms to respond to stimuli. Immediate Response to Stimulus Nastic Movements The movement of a plant in response to an external stimulus in which the direction of response is not determined by the direction of stimulus is called nastic movement. Nastic movements are shown by flat parts of the plants such as leaves and petals. Examples: 1. Daisy flowers close at dusk and open at daybreak; this may be referred to as sleep movements. 2. Mimosa pudica or the touch-me-not plant has tiny leaflets which fold up in response to mechanical stimuli such as touch, drops of rain or even a gust of wind. This quick response is due to the sudden loss of water from the cells in its swollen leaf base. This response however should not be confused with thigmotropism as the folding of leaves always occurs in the same direction irrespective of the direction of the stimulus. www.topperlearning.com 2 BIOLOGY CONTROL AND COORDINATION Photonasty is a nastic movement to the light and dark phases of the day. Examples: 1. Flowers of primrose blossom during the evening but close during the day. 2. Moon flowers close their petals in the morning when there is bright light, and they open when the light fades. Nyctinasty is the movement in response to dark. Certain parts of a plant such as the leaves and flowers take up a different posture at night than that in the day. Example: Leaves of the rain tree fold by nightfall. -
Grade 11 Biology Week 3 Lesson 1 Worksheet 1 and Solutions
MINISTRY OF EDUCATION SECONDARY ENGAGEMENT PROGRAMME GRADE 11 BIOLOGY Week 3 Lesson 1: Worksheet 1 Instructions: Circle the letter that corresponds with your chosen answer 1. Phototropism and geotropism are responses to stimuli by plants. Which of the following best represent the type of stimulus plants respond to in phototropism and geotropism respectively? (A) Photosynthesis and moisture (B) Water and light (C) Light and gravity (D) Warmth and water 2. A chemical substance that causes plants to elongate and grow cells faster from the part that is farthest from light is known as? (A) Axon (B) Chromatin (C) Autotrophs (D) Auxin 3. Growth triggered by contact in plants is known as? (A) Thermonasty (B) Nyctinasty (C) Non-directional response (D) Hyponasty 4. Growth movement produces by plants when they respond to a stimulus is known as? (A) Thigmotactic (B) Tropism (C) Hydrotropism (D) Taxic movement 5. Flies and cockroaches move away from light. This response is known as? (A) Negative phototaxis (B) Positive phototaxis (C) Negative chemotaxis (D) Positive aerotaxis 6. The main importance of responding adequately to stimuli is? (A) Reproduction (B) Survival (C) Growth (D) Adaptation Question 7 refers to the following diagram (image from biology for csec examination 3rd edition-Macmillan) 7. The diagram above represents which of the following? (A) Geotropism (B) Negative phototropism (C) Positive phototropism (D) Positive phototaxis Question 8 refers to the following diagram. 8. The diagram above accurately represents which of the following? (A) Thermonasty (B) Seismonasty (C) Hydronasty (D) Tropism Question 9 refers to the diagram below (A) (B) (C) (D) 9. -
Asymmetric Distribution of Cytokinins Determines Root Hydrotropism in Arabidopsis Thaliana
www.nature.com/cr www.cell-research.com ARTICLE OPEN Asymmetric distribution of cytokinins determines root hydrotropism in Arabidopsis thaliana Jinke Chang1, Xiaopeng Li1, Weihao Fu1, Jiawen Wang1, Yueyuan Yong1, Hongyong Shi1, Zhaojun Ding 2, Hong Kui1, Xiaoping Gou 1, Kai He1 and Jia Li1 The phenomenon of plant root tips sensing moisture gradient in soil and growing towards higher water potential is designated as root hydrotropism, which is critical for plants to survive when water is a limited factor. Molecular mechanisms regulating such a fundamental process, however, are largely unknown. Here we report our identification that cytokinins are key signaling molecules directing root growth orientation in a hydrostimulation (moisture gradient) condition. Lower water potential side of the root tip shows more cytokinin response relative to the higher water potential side. Consequently, two cytokinin downstream type-A response regulators, ARR16 and ARR17, were found to be up-regulated at the lower water potential side, causing increased cell division in the meristem zone, which allows the root to bend towards higher water potential side. Genetic analyses indicated that various cytokinin biosynthesis and signaling mutants, including the arr16 arr17 double mutant, are significantly less responsive to hydrostimulation. Consistently, treatments with chemical inhibitors interfering with either cytokinin biosynthesis or cell division completely abolished root hydrotropic response. Asymmetrically induced expression of ARR16 or ARR17 effectively led to root bending in both wild-type and miz1, a previously known hydrotropism-defective mutant. These data demonstrate that asymmetric cytokinin distribution is a primary determinant 1234567890();,: governing root hydrotropism. Cell Research (2019) 29:984–993; https://doi.org/10.1038/s41422-019-0239-3 INTRODUCTION (ahr1), respectively.7,8 Genes corresponding to the nhr and ahr1 Water distribution in soil is largely heterogeneous.1 Accord- mutants, however, have not been identified. -
Root Plasticity in the Pursuit of Water
plants Review Root Plasticity in the Pursuit of Water Hillel Fromm School of Plant Sciences and Food Security, Faculty of Life Sciences, Tel Aviv University, Tel Aviv 69978, Israel; [email protected] Received: 24 June 2019; Accepted: 19 July 2019; Published: 22 July 2019 Abstract: One of the greatest challenges of terrestrial vegetation is to acquire water through soil-grown roots. Owing to the scarcity of high-quality water in the soil and the environment’s spatial heterogeneity and temporal variability, ranging from extreme flooding to drought, roots have evolutionarily acquired tremendous plasticity regarding their geometric arrangement of individual roots and their three-dimensional organization within the soil. Water deficiency has also become an increasing threat to agriculture and dryland ecosystems due to climate change. As a result, roots have become important targets for genetic selection and modification in an effort to improve crop resilience under water-limiting conditions. This review addresses root plasticity from different angles: Their structures and geometry in response to the environment, potential genetic control of root traits suitable for water-limiting conditions, and contemporary and future studies of the principles underlying root plasticity post-Darwin’s ‘root-brain’ hypothesis. Our increasing knowledge of different disciplines of plant sciences and agriculture should contribute to a sustainable management of natural and agricultural ecosystems for the future of mankind. Keywords: drought; hydraulic lift; hydropatterning; hydrotropism; phenotypic plasticity; rhizosphere; root system architecture; xerobranching; xerotropism 1. Introduction: The Challenge of Water Acquisition and Root Phenotypic Plasticity As plants colonized the land hundreds of million years ago, replenishing their declining water supply has become one of their greatest challenges. -
Animal Behavior Types
B.Sc. Part-I Paper - II Animal Behavior: Innate Presented by : ShushmaKumari HOD & Assistant Professor, Zoology, J. D. W. College, Patna The first type is innate behavior. From Latin . innatus "inborn,“ Innate behaviors are those you develop on your own, which do not need to be taught or learned. Innate behavior is something the animal is born knowing how to do. Examples include fish swimming and geese migrating. What other examples can you think of? Innate behavior Behavior determined by the "hard-wiring" of the nervous system is Innate behavior . It is usually inflexible, a given stimulus triggering a given response. Example: A salamander raised away from water until long after its siblings begin swimming successfully will swim every bit as well as they the very first time it is placed in the water. Clearly this rather elaborate response is "built in" in the species and not something that must be acquired by practice. Types of innate behavior Irritability Tropism Nasties Taxis Kinesis Reflex action Instinct Motivation Let‘s study detail Types if innate behavior 1: Irritability def.: Irritability is an excessive response to stimuli. Conditions Irritability can occur in people experiencing any of a variety of conditions, including: Anxiety Alcoholism Fever 2: tropism : Cause of name: (from Greek trope, "a turning") Def: it is a biological phenomenon, indicating growth or turning movement of a biological organism, usually a plant, in response to an environmental In tropisms, this response is dependent on the direction of the stimulus Tropisms are typically associated with plants. Types of tropism Chemotropism, movement or growth in response to chemicals Geotropism (or gravitropism), movement or growth in response to gravity Heliotropism, movement or growth in response to sunlight Sonotropism, movement or growth in response to sound. -
Control and Co-Ordination in Plants
QUIZ: CONTROL AND CO-ORDINATION IN PLANTS 1. Movement stimulated by touch (Known as contact stimulus) is called (a) Geotropism (b) Thigmotropism (c) Phototropism (d) Chemotropism 2. The following hormone plays a role in thigmotropism (a) Gibbrellic acid (b) Cytokinin (c) Auxin (d) Abscisic acid 3. An example for chemotropism is pollen tube growth. The chemical involved in this phenomenon is (a) Zinc (b) Mercury (c) Carbon (d) Calcium 4. Among the following tropic movements which one may be sometimes independent of growth . (a) Phototropism (b) Chemotropism (c) Thigmotropism (d) Geotropism 5. What is common to phototropism and Geotropism? (a) Both can be observed in a single plant simultaneously. (b) Both are directional movements and occur in the same direction. (c) Both are triggered by environmental factors such as light. (d) Both occur only in the laboratory and not in nature. 1 6. It is commonly observed in the kitchen that freshly harvested potatoes never sprout. Only the old potatoes (potatoes harvested in the last season) sprout profusely. The possible reason could be (a) Fresh potato tubers do not have adventitious buds. (b) Fresh potato tubers contain large amount of water. (c) Fresh potato tubers contain starch only. (d) Fresh potato tubers are in dormant state and require time for ripening. 7. Motion or orientation of flowers of sunflower plant in response to direction of the sun is an example for (a) Thigmotropism (b) Phototropism (c) Heliotropism (d) Hydrotropism Answers: 1. (b) Explanation: The term Thigmotropism refers to movement stimulated by touch. Geotropism, Phototropism, Chemotropism refers to movement stimulated by gravitational force, light and chemical respectively. -
Hydrotropism
View metadata, citation and similar papers at core.ac.uk brought to you by CORE provided by Repository@Nottingham 1 Hydrotropism – How Roots Search for Water 2 3 Daniela Dietrich 4 5 Centre for Plant Integrative Biology and Plant & Crop Sciences, School of 6 Biosciences, University of Nottingham, Nottingham, LE12 5RD, UK 7 [email protected] 8 Tel: 01159516108 9 10 Submitted 03/10/2017 11 Resubmitted 19/12/2017 12 13 1 table 14 3 figures (all colour) 15 7063 words 16 1 17 Hydrotropism – How Roots Search for Water 18 19 Abstract 20 Fresh water is an increasingly scarce resource for agriculture. Plant roots mediate 21 water uptake from the soil and have developed a number of adaptive traits like 22 hydrotropism to aid water foraging. Hydrotropism modifies root growth to respond to 23 a water potential gradient in soil and grow towards areas with a higher moisture 24 content. Abscisic acid (ABA) and a small number of genes, including ABA signal 25 transducers, MIZ2/GNOM and the hydrotropism-specific MIZ1 are known to be 26 necessary for the response in Arabidopsis thaliana, whereas the role of auxin in 27 hydrotropism appears to vary depending on the plant species. This review will 28 describe recent progress characterising the hormonal regulation of hydrotropism. 29 Recent advances in identifying the sites of hydrotropic perception and response, 30 together with its interaction with gravitropism, will also be discussed. Finally, I will 31 describe putative mechanisms for perception of the water potential gradient and a 32 potential role for hydrotropism in acclimatising plants to drought conditions. -
Biology 101 Flower Parts
Biology 101 Fall, 2008 Week 3 – Flowers Flower parts Flower parts are arranged in 4 whorls 1. The gynoecium (female) 1 stigma 1. The gynoecium (female) pistil style carpel ovary Flower parts are arranged in 4 whorls 2. The androecium (male) Flower parts are arranged in 4 whorls 2. The androecium (male) stamen anther filament 2 Flower parts are arranged in 4 whorls 1. The gynoecium (female) 2. The androecium (male) 3. The corolla (petals) Flower parts are arranged in 4 whorls 3. The corolla (petals) Flower parts are arranged in 4 whorls 1. The gynoecium (female) 2. The androecium (male) 3. The corolla (petals) 4. The calyx (sepals) 3 4. The calyx (sepals) Flower parts are arranged in 4 whorls 1. The gynoecium (female) 2. The androecium (male) 3. The corolla (petals) 4. The calyx (sepals) All four whorls are attached to the receptacle corolla androecium receptacle gynoecium calyx 4 stigma anther style pistil stamen (gynoecium) (androecium) filament ovary (carpels) ovule petals (corolla) receptacle sepals (calyx) corolla peduncle perianth Flowers FLOWERS, FRUITS and SEEDS Angiosperms: vessel-seeded vessel: gynoecium carpel ovary (pod) 5 Central cell nuclei Inflorescence Types 6 Gynoecium Perigynous receptacle Ovules attached to placenta of ovary Placentation : pattern of ovule free attachment central axile parietal CARPEL Carpel derived evolutionarily from leaves Gynoecium types: Simple Complex 7 OVULE ovule functions as: megasporangium megagametophyte embryo sac MEGASPOROGENESIS ovule / megasporangium nucellus megaspore mother