Determination of Single Base Mutations Related to the Gene Specific Diseases by Using Electrochemical Dna Biosensors in the Integrated System
Total Page:16
File Type:pdf, Size:1020Kb
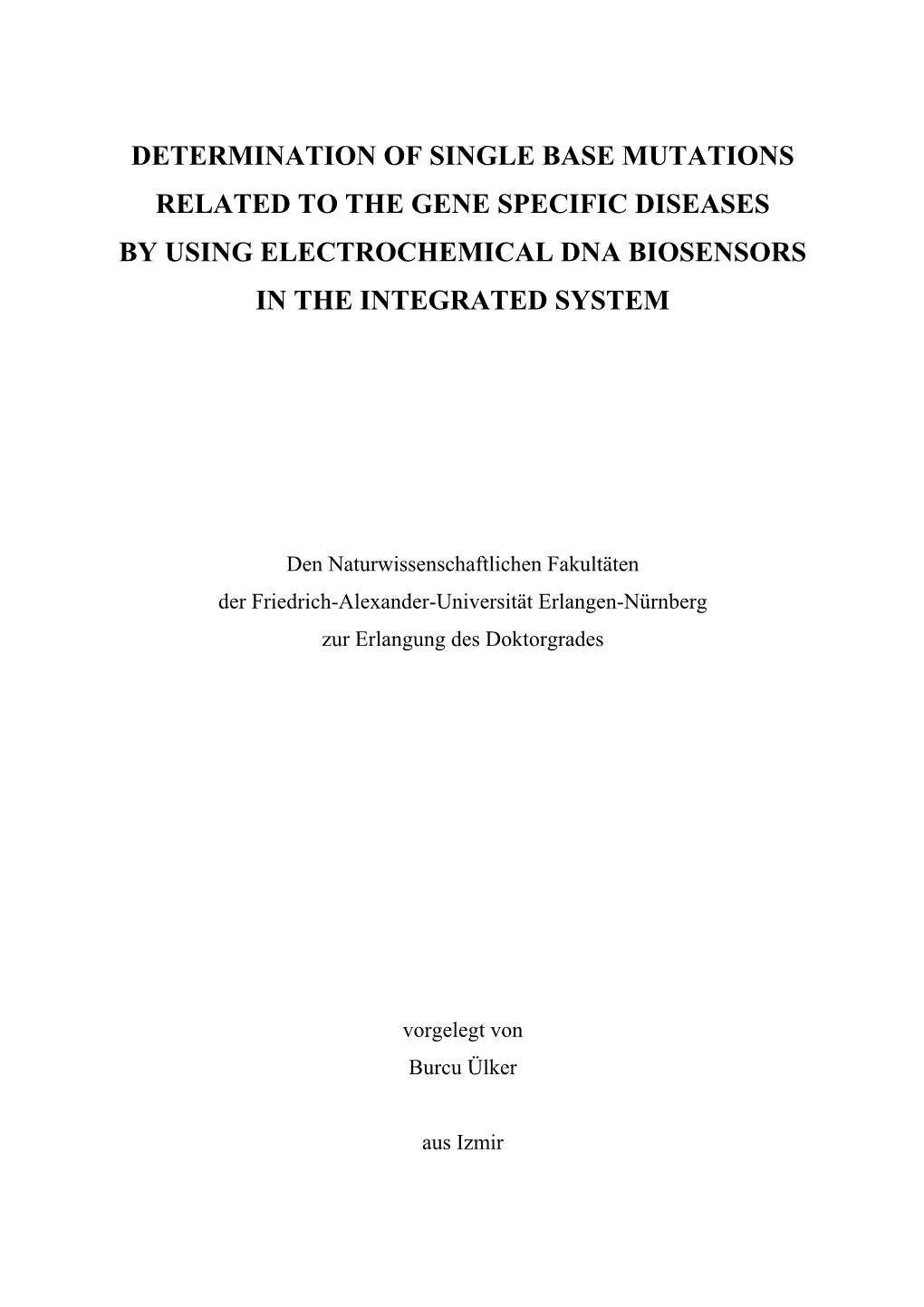
Load more
Recommended publications
-
Cell Notation Practical Galvanic Cells -Batteries
Basic Redox Vocabulary • Write reactions for each of the following: • oxidation of metallic nickel by BiO+ • reduction of Zn2+ by hydroxide ion • reaction of Fe 2+ with Hg2+ 2+ • reaction of Cd with NO2 • AgI acting as an oxidizing agent toward Sn 2+ • What’s wrong with • The oxidation of Cr by Cl- • The reduction of Co 2+ by Ag+ Cell Notation • As was noted earlier, galvanic cells normally consist of two distinct regions, one housing the oxidation half and the other the reduction half. There is a simplified notation form that allows one to represent the cell easily( text p 798- 799). • The oxidation is written on the left and the reduction on the right. starting with the anode material and ending with the cathode material. • phase boundaries represented with single vertical lines “ |” • the physical separation between the two half cells is a double v ertical line “||” if it’s a salt bridge and with a single broken vertical line, “!”, if it’s a liquid junction • within each have cell, the species are written in a reactant-product order, separated by commas if they are in the same phase. Acid/base components should be included • The electrode material may be actively participating in the redox chemistry (active electrode) or merely providing surface for the electron transfer (passive or inert electrode, usually graphite or Pt) • Represent the following as galvanic cells(assume the reactions are spontaneous as written) • Tl(s) + Cd 2+ ó Tl + + Cd(s) - 2+ 2+ • Pb(s) + MnO4 ó Pb + Mn (acid) 2+ 4+ • O2(g) + Sn ó H2O + Sn Practical Galvanic Cells -batteries • Batteries represent the most common application of the electrochemical cell. -
Voltaic Cells
Voltaic Cells Tro Chapter 19 – Electrochemistry 19.3 Voltaic(or Galvanic) Cells: Generating Electricity from Spontaneous Chemical Reactions Electric Current Flowing Directly Between Atoms Tro, Chemistry: A Molecular Approach 2 Electrochemical Cells Voltaic (Galvanic) ΔG < 0 to Electrolytic Δ G > 0 uses electrical generate electrical energy. energy to drive non-spontaneous process. Electrochemical Cells • Oxidation and reduction half-reactions are kept separate in half-cells. • Electron flow through a wire along with ion flow through a solution constitutes an electric circuit. • It requires a conductive solid electrode to allow the transfer of electrons. – Through external circuit – Metal or graphite • Requires ion exchange between the Daniell Cell two half-cells of the system. – Electrolyte Definitions Anode Salt Bridge • Electrode where oxidation always occurs • An inverted, U-shaped tube containing a • More negatively charged electrode in strong electrolyte and connecting the two voltaic cell half-cells. • Typically made of metal that is oxidized Cathode • Electrode where reduction always occurs Potential Difference • More positively charged electrode in • The difference in potential energy voltaic cell between the reactants and products. • Typically metal that is produced by reduction (Caused by an electric field resulting from the charge difference on the two If the redox reaction involves the oxidation or electrodes.) reduction of an ion to a different oxidation state, or the oxidation or reduction of a gas, we Cell Potential (Ecell or emf) may use an inert electrode. • The potential difference between the anode and the cathode in a voltaic cell. • An inert electrode is one that not does participate in the reaction but just provides a surface on which the transfer of electrons can take place. -
Galvanic Cell Notation • Half-Cell Notation • Types of Electrodes • Cell
Galvanic Cell Notation ¾Inactive (inert) electrodes – not involved in the electrode half-reaction (inert solid conductors; • Half-cell notation serve as a contact between the – Different phases are separated by vertical lines solution and the external el. circuit) 3+ 2+ – Species in the same phase are separated by Example: Pt electrode in Fe /Fe soln. commas Fe3+ + e- → Fe2+ (as reduction) • Types of electrodes Notation: Fe3+, Fe2+Pt(s) ¾Active electrodes – involved in the electrode ¾Electrodes involving metals and their half-reaction (most metal electrodes) slightly soluble salts Example: Zn2+/Zn metal electrode Example: Ag/AgCl electrode Zn(s) → Zn2+ + 2e- (as oxidation) AgCl(s) + e- → Ag(s) + Cl- (as reduction) Notation: Zn(s)Zn2+ Notation: Cl-AgCl(s)Ag(s) ¾Electrodes involving gases – a gas is bubbled Example: A combination of the Zn(s)Zn2+ and over an inert electrode Fe3+, Fe2+Pt(s) half-cells leads to: Example: H2 gas over Pt electrode + - H2(g) → 2H + 2e (as oxidation) + Notation: Pt(s)H2(g)H • Cell notation – The anode half-cell is written on the left of the cathode half-cell Zn(s) → Zn2+ + 2e- (anode, oxidation) + – The electrodes appear on the far left (anode) and Fe3+ + e- → Fe2+ (×2) (cathode, reduction) far right (cathode) of the notation Zn(s) + 2Fe3+ → Zn2+ + 2Fe2+ – Salt bridges are represented by double vertical lines ⇒ Zn(s)Zn2+ || Fe3+, Fe2+Pt(s) 1 + Example: A combination of the Pt(s)H2(g)H Example: Write the cell reaction and the cell and Cl-AgCl(s)Ag(s) half-cells leads to: notation for a cell consisting of a graphite cathode - 2+ Note: The immersed in an acidic solution of MnO4 and Mn 4+ reactants in the and a graphite anode immersed in a solution of Sn 2+ overall reaction are and Sn . -
Electrochemistry –An Oxidizing Agent Is a Species That Oxidizes Another Species; It Is Itself Reduced
Oxidation-Reduction Reactions Chapter 17 • Describing Oxidation-Reduction Reactions Electrochemistry –An oxidizing agent is a species that oxidizes another species; it is itself reduced. –A reducing agent is a species that reduces another species; it is itself oxidized. Loss of 2 e-1 oxidation reducing agent +2 +2 Fe( s) + Cu (aq) → Fe (aq) + Cu( s) oxidizing agent Gain of 2 e-1 reduction Skeleton Oxidation-Reduction Equations Electrochemistry ! Identify what species is being oxidized (this will be the “reducing agent”) ! Identify what species is being •The study of the interchange of reduced (this will be the “oxidizing agent”) chemical and electrical energy. ! What species result from the oxidation and reduction? ! Does the reaction occur in acidic or basic solution? 2+ - 3+ 2+ Fe (aq) + MnO4 (aq) 6 Fe (aq) + Mn (aq) Steps in Balancing Oxidation-Reduction Review of Terms Equations in Acidic solutions 1. Assign oxidation numbers to • oxidation-reduction (redox) each atom so that you know reaction: involves a transfer of what is oxidized and what is electrons from the reducing agent to reduced 2. Split the skeleton equation into the oxidizing agent. two half-reactions-one for the oxidation reaction (element • oxidation: loss of electrons increases in oxidation number) and one for the reduction (element decreases in oxidation • reduction: gain of electrons number) 2+ 3+ - 2+ Fe (aq) º Fe (aq) MnO4 (aq) º Mn (aq) 1 3. Complete and balance each half reaction Galvanic Cell a. Balance all atoms except O and H 2+ 3+ - 2+ (Voltaic Cell) Fe (aq) º Fe (aq) MnO4 (aq) º Mn (aq) b. -
3297 Chapter 13.Indd
Inert Electrodes The zinc anode and copper cathode of a Daniell cell and the silver and chromium electrodes in Figure 13.4 are all metals and can act as electrical conductors. However, some redox reactions involve oxidizing and reducing agents that are not solid metals but, instead, are dissolved electrolytes or gases and, therefore, cannot be used as electrodes. To construct a voltaic cell that will use these oxidizing and reducing agents, you have to use inert electrodes. An inert electrode is an electrode made from a material that is neither a reactant nor a product of the redox reaction. Instead, the inert electrode can carry a current and provide a surface on which redox reactions can occur. Figure 13.5 shows a cell that contains one example of an inert electrode—a platinum electrode. The complete balanced equation, net ionic equation, and half-reactions for this cell are given below. complete balanced equation: Pb(s) + 2FeCl3(aq) → 2FeCl2(aq) + PbCl2(aq) net ionic equation: Pb(s) + 2Fe3+(aq) → 2Fe2+(aq) + Pb2+(aq) oxidation half-reaction: Pb(s) → Pb2+(aq) + 2e– reduction half-reaction: Fe3+(aq) + e– → Fe2+(aq) The anode is the lead electrode. Lead atoms lose electrons that remain in the electrode while the lead(II) ions dissolve in the solution in the same way that the anode did in previous example. However, the reduction half-reaction involves iron(III) ions that accept an electron from the platinum inert electrode and become iron(II) ions. The platinum atoms in the electrode (cathode) remain unchanged. Voltmeter e- e- Anode Salt bridge Cathode (-) K+ (+) Pb Cl- Pt 2e- e- Fe3+ Pb Fe2+ Pb2+ PbCl2 FeCl3 FeCl2 Figure 13.5 This cell uses an inert electrode to conduct electrons. -
Au(Hkl): Surface X-Ray Diffraction Studies at the Electrochemical Interface
Au(hkl): Surface X-ray Diffraction Studies at the Electrochemical Interface By Joshua James Fogg Oliver Lodge Laboratory, Department of Physics University of Liverpool Thesis submitted in accordance with the requirements of the University of Liverpool for the degree of Doctor in Philosophy September 2018 i Abstract In-situ surface x-ray diffraction (SXRD) measurements have been performed to develop an increased understanding of electrocatalytic reactions and electrodeposition processes occurring on gold single crystal surfaces. The surfaces of gold exhibit a rich physical behaviour that is interesting not only from a structural perspective but also for applications in areas such as heterogeneous catalysis and electrocatalysis. The surface reconstructions of Au(111) and Au(100) have been found to undergo a potential dependent in plane surface compression in alkaline solution that is remarkably similar despite the underlying geometry. The compressibility is linked to the charge on the surface Au atoms with a simple free electron model. The surface compression and the reversible lifting of the reconstructions are determined by the interplay between surface charge and the adsorption of hydroxide species. Carbon monoxide adsorption is shown to supress both the potential-induced changes in surface compression and the lifting of the reconstruction leading to the promotion of electrocatalytic reactivity. Measurements of the Au(111)/Pb UPD system in acidic solution reveal a substitutional Au/Pb surface alloy of the ratio ~ 4:1 that forms at intermediate coverages of Pb during the stripping of Pb from the Au(111) electrode surface. Investigations into the adsorption of Acetonitrile (AcN) on the Au(111) electrode surface in sulphuric acid solution find that AcN has an enhancing effect on the adsorption of sulphate molecules. -
Novel Non-Aqueous Symmetric Redox Materials for Redox Flow Battery Energy Storage
Novel Non-Aqueous Symmetric Redox Materials for Redox Flow Battery Energy Storage Craig G. Armstrong This dissertation is submitted for the degree of Doctor of Philosophy January 2020 Department of Chemistry The search for ‘electrochemically promiscuous’ redox materials… - Craig Armstrong, 2017 ii Declaration This thesis has not been submitted in support of an application for another degree at this or any other university. It is the result of my own work and includes nothing that is the outcome of work done in collaboration except where specifically indicated. Many of the ideas in this thesis were the product of discussion with my supervisor Dr Kathryn E. Toghill. Dr Ross W. Hogue assisted in the acquisition of experimental results in chapters 4, 6 and 7. He is also credited for co-writing [3], of which Chapter 6 is based, and is a second author on [4]. Excerpts of this thesis have been published in the following academic publications [1–4]. [1] C.G. Armstrong, K.E. Toghill, Cobalt(II) complexes with azole-pyridine type ligands for non-aqueous redox-flow batteries: Tunable electrochemistry via structural modification, J. Power Sources. 349 (2017) 121–129. doi:10.1016/j.jpowsour.2017.03.034. [2] C.G. Armstrong, K.E. Toghill, Stability of molecular radicals in organic non- aqueous redox flow batteries: A mini review, Electrochem. Commun. 91 (2018) 19–24. doi:10.1016/j.elecom.2018.04.017. [3] R. Hogue, C. Armstrong, K. Toghill, Dithiolene Complexes of First Row Transition Metals for Symmetric Non-Aqueous Redox Flow Batteries, ChemSusChem. (2019) 1–11. doi:10.1002/cssc.201901702. -
Galvanic and Electrolytic Cells
2014/01/01 Difference between galvanic & electrolytic cells Galvanic cells consist of self sustaining electrode reactions converting chemical energy into electrical Galvanic – no batteries energy. GALVANIC AND Galvanic & electrolytic cells They produce electricity ELECTROLYTIC CELLS Electrolytic cells are sustained by a supply of electrical energy from a current source, converting electrical energy into chemical energy. They are used to electroplate items. Electrolytic – batteries required Lemon battery ZnO - Redox reaction REDOX REACTIONS Mg → Mg2+ + 2e- oxidation reaction OXIDATION REDUCTION reducing agent (donates electrons and so can cause reduction) - - Cl2 + 2e → 2Cl reduction reaction A reaction in A reaction in which a which a oxidising agent (accepts electrons and substance substance gains so can cause oxidation) Redox agents loses electrons electrons Mg → Mg2+ + 2e- Mg is oxidised (1) - - Cl2 + 2e → 2Cl Cl2 is reduced (2) Mg + Cl2 → MgCl2 Redox reaction Mg in Cl2 The electrons cancel each other out. Redox reactions 2+ - MgCl2 is an ionic compound (Mg 2Cl ) Gain & loss of electrons The equation shows 2 half reactions (1 and Redox examples 2) that add to give the full redox reaction. 1 2014/01/01 DIRECT ELECTRON TRANSFER From the observations we can infer that: Cu in AgNO3 Cu → Cu2+ + 2e- A coil of copper was Ag+ + e- → Ag placed in a silver nitrate solution Electrons are transferred The solution became blue from the copper atoms on because copper ions the piece of copper, to the were formed. silver ions in the silver nitrate solution. Solid silver deposited on This is a redox reaction. Cu in AgNO3 the copper wire. This is a spontaneous reaction. -
Table of Contents
THE UNIVERSITY OF HULL ELECTRO-CATALYTIC REACTIONS Being a Thesis submitted for the Degree of Doctor of Philosophy THE UNIVERSITY OF HULL by Carlos Lledo-Fernandez (June 2009) Declaration The work described in this thesis was carried out in the Department of Chemistry, University of Hull under the supervision of Professor G.M. Greenway and Dr. J. Wadhawan between October 2004 and September 2008. Except where indicated by references, the work is original and has not been submitted for any other degree. Carlos Lledo-Fernandez June 2009 2 Table of Contents TITLE 1 DECLARATION 2 TABLE OF CONTENTS 3 ACKNOWLEDGEMENTS……………………………………………………. 8 ABSTRACT……………………………………………………………………... 10 CHAPTER 1-INTRODUCTION……………………………………………… 13 1 AIMS OF THE THESIS……………………………………………………………... 14 1.1 DYNAMIC ELECTROCHEMISTRY………………………………………….. 14 1.1.1 INTRODUCTION…………………………………………………………………….. 14 1.1.2 THE INTERFACIAL REGION AND ELECTROLYTE DOUBLE LAYER………... 15 1.2 ELECTROCHEMICAL CELL AND REACTIONS……………………………. 17 1.3 CELL DESIGN AND ELECTRODE PLACEMENT……………………………. 20 1.4 MATERIAL TRANSPORT……………………………………………………….. 21 1.4.1 DIFFUSION…………………………………………………………………………….. 23 1.4.2 CONVECTION………………………………………………………………………... 25 1.4.3 MIGRATION………………………………………………………………………….. 27 1.5 CYCLIC VOLTAMMETRY……………………………………………………… 27 1.5.1 THEORY AND APPLICATIONS OF THE CYCLIC VOLTAMMETRY FOR 29 MEASURAMENT OF ELECTRODE REACTIONS KINETICS……………………………….. 1.5.2 CYCLIC VOLTAMMETRY AT PLANAR ELECTRODES………………………… 29 1.5.2.1 CYCLIC VOLTAMMETRY WITH REVERSIBLE SYSTEM………………………………………. 30 1.5.2.2 CYCLIC VOLTAMMETRY WITH IRREVERSIBLE SYSTEM……………………………………. 32 1.5.2.3 CYCLIC VOLTAMMTRY WITH QUASI-REVERSIBLE SYSTEM……………………………….. 34 1.5.2.4 COMPARISON BETWEEN REVERSIBLE AND IRREVERSIBLE VOLTAMMOGRAMS……… 36 1.6 AMPEROMETRIC DETECTION………………………………………………... 37 1.7 POTENTIAL STEP : CHRONOAMPEROMETRY…………………………….. 37 1.8 HYDRODYNAMIC ELECTRODES……………………………………………... 39 1.8.1 LIMITING CURRENTS………………………………………………………………. -
Electrochemistry Chem 35.5
Announcements Problems Chapter 21: 2,9,10,11,13,17,22,29,31,38,40,44,46,50,53,58,62,64,65,70, 72,73,82,85,87 Wonderful job with the presentations I was very impressed with all of them. Exam 3 March 17 Comprehensive Final Exam: March 24 7:30AM - 9:30 C114 This is an electrochemical cell, also called a voltaic or galvanic cell. Chemists name parts electrochemical cell names. Salt bridge with non-reacting Electron but conductive salt to replace - -------> lost electrons and reduced Anode (-): Flow e cations Oxidation always occurs here Cathode (+): Reduction always - + occurs here. Anode Cathode Electrolyte Electrolyte Example Problem: Making a Voltaic Cell Diagram Diagram, show balanced equations, and write the notation for a voltaic cell that consists of one half-cell with a Cr solid electrode in a Cr(NO3)3 solution, another half-cell with an Ag solid electrode in an AgNO3 solution, connected by a KNO3 salt bridge. What would the cell potential be under standard state conditions? 3+ 0.74 = Cr + 2 e− Cr(s) − −−→ Example Problem: Making a Voltaic Cell Diagram Cr(s) | Cr3+(aq) || Ag+(aq) | Ag(s) oxidation half-reaction: Cr(s) Cr3+(aq) + 3e- reduction half-reaction: Ag+(aq) + e- Ag(s) Cr(s) + Ag+(aq) Cr3+(aq) + Ag(s) Galvanic cells can also be made using “non-active electrode”, or electrodes that don’t undergo reaction directly in the cell. The non-active electrodes are typically carbon or platinum. reduction half-reaction: - + - MnO4 (aq) + 8H (aq) + 5e oxidation half-reaction: 2+ Mn (aq) + 4H2O(l) - - 2I (aq) I2(s) + 2e overall (cell) reaction: - + - 2+ 2MnO4 (aq) + 16H (aq) + 10I (aq) 2Mn (aq) + 5I2(s) + 8H2O(l) We have and use standard cell notation for non- active electrodes too as shown below. -
Investigation of the Copper Metallic Content in Plasma Activated Water from the Electrode Erosion in a Pin-To-Liquid Discharge System
Investigation of the Copper Metallic Content in Plasma Activated Water from the Electrode Erosion in a Pin-to-Liquid discharge system Simon Nicolas Dib McGill University Montreal QC, Canada August 2020 A thesis submitted to McGill University in partial fulfillment of the requirements for the degree of Master of Engineering © Simon Nicolas Dib, 2020 Abstract When it comes to non-thermal plasmas, many questions are, to this day, left unanswered primarily because of the many diverse configurations they can adopt. Due to their extremely small mass, electrons are inefficient at transferring kinetic energy to heavy particles and ultimately to the walls confining the plasma volume. Therefore, non-thermal plasmas implement high reactivity environments at approximately room temperature, which is very advantageous especially when it comes to treating temperature-sensitive materials. Specifically, in a pin-to-water non-thermal plasma configuration, chemical reactions and physical morphologies that are unconventional at room temperature become realized, such as the generation of reactive oxygen and/or nitrogen species (RONS) and the induction of cathodic erosion. Unlike RONS, cathodic erosion in a pin-to-water plasma system has almost been completely unventured. It proposes a method of metal deposition into water and creates a synergistic effect due to its coexistence with RONS. The synergistic effect applies to the plasma activated water (PAW), whereby aqueous metallic ions and solid metallic particles are deposited as a result of erosion caused by plasma discharges over a water surface. In order to comprehend the role metals play, the goal of this thesis has been to characterize the metal content of PAW and study the effect of different plasma atmospheres on the levels of erosion, which are correlated to metal deposition. -
Teacher's Tools® Chemistry
Teachers Tools® Chemistry Electrochemistry: Voltaic Cells: Student Review Notes Cell Voltages: Standard Cell Potentials The value of the Cell Voltage or Reaction Potential (they mean the same thing) is the driving force behind the redox reaction. Cell voltage depends upon (1) the nature of the redox reaction (2) the concentration of the species involved in the reaction Standard reduction potentials have been tabulated for the reduction of many of the species you will see involved in redox reactions. Note: only the reduction potential (Eºred) is given on these tables. The potential associated with the reverse reaction, the oxidation potential (Eºox) is the negative of Eºred. Also, if the reaction you are examining is a multiple of the one you find on the table, DO NOT multiply Eºred by the associated constant. Potential is like the height of a waterfall, the number of electrons you flow over the waterfall does not change the height of the waterfall. Standard means: 1 M solutions of ions or molecules and a pressure of 1 atm. Eºox + Eºred = Eºtotal = the standard potential for the redox reaction The tabulated half-reaction redox potentials are measured in reference to the reduction of hydrogen cation. This is the arbitrary zero point established for the reduction potential scale. + - 2 H (1 M) + 2 e H2(g) Eºred = 0.00 V Determining Eºtot for a redox reaction. iron goes from +3 to +2 that is a gain of electrons or 3+ - 2+ reduction Example: 2 Fe (aq) + 2 I (aq) 2 Fe (aq) + I2(s) iodine goes from -1 to 0 that is a loss of electrons or oxidation 3+ - 2+ reduction: 2 Fe + 2 e 2 Fe Eºred = 0.77 V go to the table of standard reduction potentials and find the reactions.