Control of Slc7a5 Sensitivity by the Voltage-Sensing Domain of Kv1
Total Page:16
File Type:pdf, Size:1020Kb
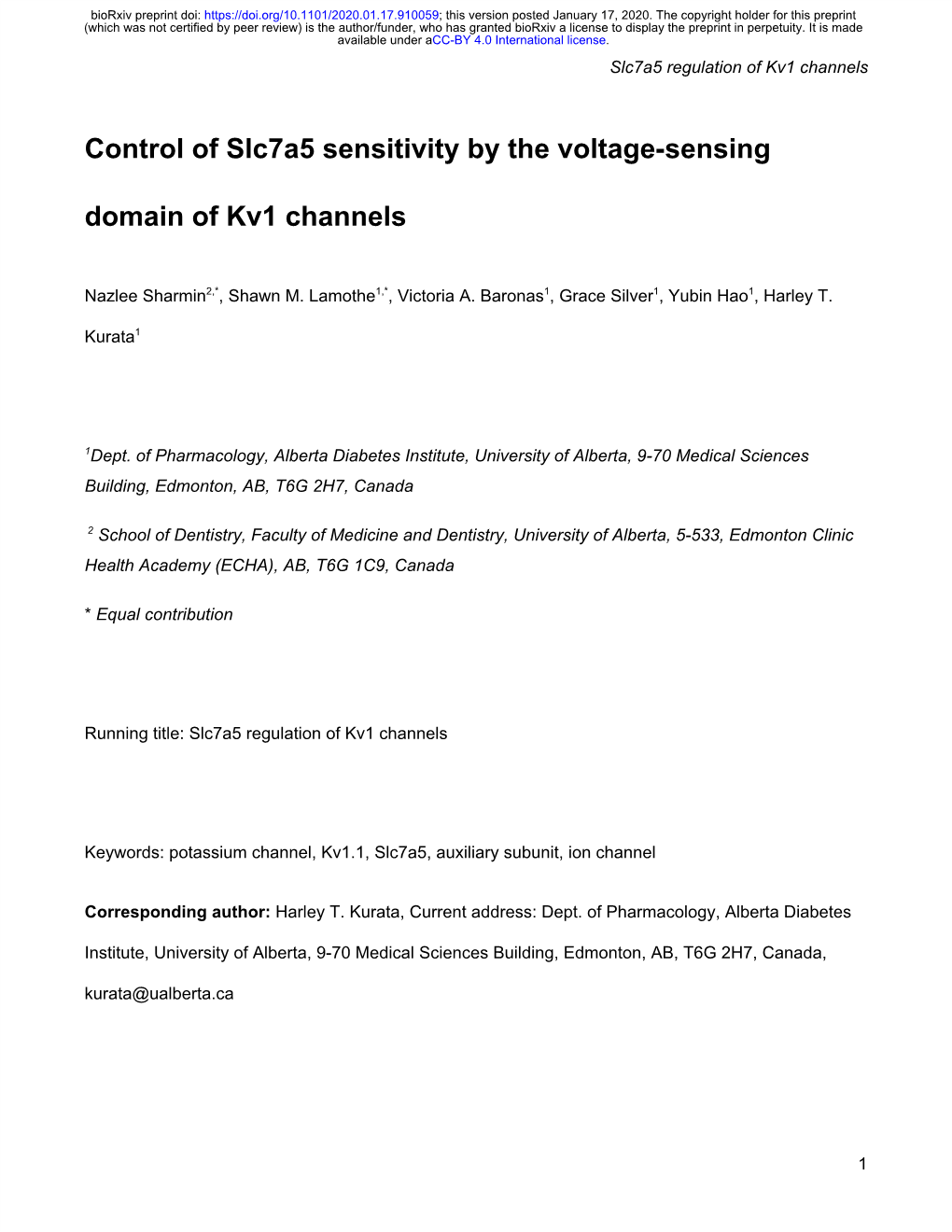
Load more
Recommended publications
-
LAT2 Monoclonal Antibody, Clone NAP-07 (PE)
LAT2 monoclonal antibody, clone NAP-07 (PE) Catalog # : MAB4522 規格 : [ 100 ug ] List All Specification Application Image Product Mouse monoclonal antibody raised against partial recombinant LAT2. Western Blot Description: Immunocytochemistry Immunogen: Recombinant protein corresponding to partial human LAT2. Immunoprecipitation Flow Cytometry Host: Mouse Theoretical MW 25-30 (kDa): Reactivity: Human, Mouse Specificity: This antibody reacts with Non-T cell activation linker (NTAL), also known as LAB (linker of activated B cells), a 25-30 KDa transmembrane adaptor protein present in membrane microdomains (rafts) of B lymphocytes, NK cells and myeloid cells. Form: Liquid Conjugation: PE Concentration: 0.1 mg/mL Isotype: IgG1 Recommend Flow Cytometry (1:30) Usage: The optimal working dilution should be determined by the end user. Storage Buffer: In PBS (0.2% BSA, 0.09% sodium azide) Storage Store in the dark at 4°C. Do not freeze. Instruction: Avoid prolonged exposure to light. Aliquot to avoid repeated freezing and thawing. Note: This product contains sodium azide: a POISONOUS AND HAZARDOUS SUBSTANCE which should be handled by trained staff only. Datasheet: Download Publication Reference 1. Topography of signaling molecules as detected by electron microscopy on plasma membrane sheets isolated from non-adherent mast cells. Lebduska P, Korb J, Tumova M, Heneberg P, Draber P.J Immunol Methods. 2007 Dec 1;328(1-2):139-51. Epub 2007 Sep 18. 2. Negative regulation of mast cell signaling and function by the adaptor LAB/NTAL. Volna P, Lebduska P, Draberova L, Simova S, Heneberg P, Boubelik M, Bugajev V, Malissen B, Wilson BS, Horejsi V, Malissen M, Draber P.J Exp Med. -
Structure-Function Study of Heterodimeric Amino Acid Transporter, LAT1-Cd98hc
Structure-function study of heterodimeric amino acid transporter, LAT1-CD98hc GEORGE NYASHA CHIDUZA Thesis submitted in accordance with the requirements of the University of Liverpool for the degree of Doctor in Philosophy February 2019 Institute of Integrative Biology University of Liverpool United Kingdom Acknowledgements Firstly, I am grateful to my supervisory team Professor S. Samar Hasnain, Dr Svetlana Antonyuk and Dr Gareth S. A. Wright, in many ways they have gone above and beyond their responsibilities toward me as required by the university, in order to help me to this position. Professor Hasnain believes in my potential and has provided me the environment, mentorship and professional network to realise it as far as I have over the course of my PhD. Dr Antonyuk has given me essential support and guidance on theoretical as well as practical aspects throughout my research. Dr Wright has also mentored and taught me much about how to think about scientific questions, and being, not just a good but efficient experimentalist. He has been a good friend to me, supporting me in important aspects of life, without which I could not be where I am today. I am grateful to Dr David Dickens who I worked with in the first two years of my PhD. He taught me the importance of keeping abreast with the scientific literature, and how to leverage that knowledge to drive my own research. He demonstrated to me a level of diligence I aspire to. I would like to acknowledge the support Professor Sir Munir Pirmohamed in the early part of my PhD, where I worked under his supervision in the Wolfson Centre for Personalised Medicine, here in Liverpool. -
Genomic Rearrangements of the 7Q11-21 Region
GENOMIC REARRANGEMENTS IN HUMAN AND MOUSE AND THEIR CONTRIBUTION TO THE WILLIAMS-BEUREN SYNDROME PHENOTYPE. by Edwin James Young A thesis submitted in conformity with the requirements for the degree of Doctor of Philosophy, Institute of Medical Science University of Toronto © Copyright by Edwin James Young (2010) Genomic rearrangements in human and mouse and their contribution to the Williams- Beuren Syndrome phenotype. Doctor of Philosophy (2010) Edwin James Young Institute of Medical Science University of Toronto Abstract: Genomic rearrangements, particularly deletions and duplications, are known to cause many genetic disorders. The chromosome 7q11.23 region in humans is prone to recurrent chromosomal rearrangement, due to the presence of low copy repeats that promote non-allelic homologous recombination. The most well characterized rearrangement of 7q11.23 is a hemizygous 1.5 million base pair (Mb) deletion spanning more than 25 genes. This deletion causes Williams-Beuren Syndrome (WBS; OMIM 194050), a multisystem developmental disorder with distinctive physical and behavioural features. Other rearrangements of the region lead to phenotypes distinct from that of WBS. Here we describe the first individual identified with duplication of the same 1.5 Mb region, resulting in severe impairment of expressive language, in striking contrast to people with WBS who have relatively well preserved language skills. We also describe the identification of a new gene for a severe form of childhood epilepsy through the analysis of individuals with deletions on ii chromosome 7 that extend beyond the boundaries typical for WBS. This gene, MAGI2, is part of the large protein scaffold at the post-synaptic membrane and provides a new avenue of research into both the molecular basis of infantile spasms and the development of effective therapies. -
The Human Gene Connectome As a Map of Short Cuts for Morbid Allele Discovery
The human gene connectome as a map of short cuts for morbid allele discovery Yuval Itana,1, Shen-Ying Zhanga,b, Guillaume Vogta,b, Avinash Abhyankara, Melina Hermana, Patrick Nitschkec, Dror Friedd, Lluis Quintana-Murcie, Laurent Abela,b, and Jean-Laurent Casanovaa,b,f aSt. Giles Laboratory of Human Genetics of Infectious Diseases, Rockefeller Branch, The Rockefeller University, New York, NY 10065; bLaboratory of Human Genetics of Infectious Diseases, Necker Branch, Paris Descartes University, Institut National de la Santé et de la Recherche Médicale U980, Necker Medical School, 75015 Paris, France; cPlateforme Bioinformatique, Université Paris Descartes, 75116 Paris, France; dDepartment of Computer Science, Ben-Gurion University of the Negev, Beer-Sheva 84105, Israel; eUnit of Human Evolutionary Genetics, Centre National de la Recherche Scientifique, Unité de Recherche Associée 3012, Institut Pasteur, F-75015 Paris, France; and fPediatric Immunology-Hematology Unit, Necker Hospital for Sick Children, 75015 Paris, France Edited* by Bruce Beutler, University of Texas Southwestern Medical Center, Dallas, TX, and approved February 15, 2013 (received for review October 19, 2012) High-throughput genomic data reveal thousands of gene variants to detect a single mutated gene, with the other polymorphic genes per patient, and it is often difficult to determine which of these being of less interest. This goes some way to explaining why, variants underlies disease in a given individual. However, at the despite the abundance of NGS data, the discovery of disease- population level, there may be some degree of phenotypic homo- causing alleles from such data remains somewhat limited. geneity, with alterations of specific physiological pathways under- We developed the human gene connectome (HGC) to over- come this problem. -
LAT2 Monoclonal Antibody, Clone NAP-07 (PE)
LAT2 monoclonal antibody, clone Storage Instruction: Store in the dark at 4°C. Do not NAP-07 (PE) freeze. Avoid prolonged exposure to light. Catalog Number: MAB4522 Aliquot to avoid repeated freezing and thawing. Regulatory Status: For research use only (RUO) Entrez GeneID: 7462 Product Description: Mouse monoclonal antibody Gene Symbol: LAT2 raised against partial recombinant LAT2. Gene Alias: HSPC046, LAB, NTAL, WBSCR15, Clone Name: NAP-07 WBSCR5, WSCR5 Immunogen: Recombinant protein corresponding to Gene Summary: This gene is one of the contiguous partial human LAT2. genes at 7q11.23 commonly deleted in Williams syndrome, a multisystem developmental disorder. This Host: Mouse gene consists of at least 14 exons, and its alternative splicing generates 3 transcript variants, all encoding the Theoretical MW (kDa): 25-30 same protein. [provided by RefSeq] Reactivity: Human,Mouse References: 1. Topography of signaling molecules as detected by Applications: Flow Cyt, ICC, IP, WB electron microscopy on plasma membrane sheets (See our web site product page for detailed applications isolated from non-adherent mast cells. Lebduska P, Korb information) J, Tumova M, Heneberg P, Draber P. J Immunol Methods. 2007 Dec 1;328(1-2):139-51. Epub 2007 Sep Protocols: See our web site at 18. http://www.abnova.com/support/protocols.asp or product 2. Negative regulation of mast cell signaling and function page for detailed protocols by the adaptor LAB/NTAL. Volna P, Lebduska P, Draberova L, Simova S, Heneberg P, Boubelik M, Specificity: This antibody reacts with Non-T cell Bugajev V, Malissen B, Wilson BS, Horejsi V, Malissen activation linker (NTAL), also known as LAB (linker of M, Draber P. -
Amino Acids: Basolateral Efflux and Extracellular Homeostasis Control Invivo
Zurich Open Repository and Archive University of Zurich Main Library Strickhofstrasse 39 CH-8057 Zurich www.zora.uzh.ch Year: 2012 Amino Acids: Basolateral efflux and extracellular homeostasis control invivo Mariotta, Luca Abstract: In all living organisms, amino acids (AA) are essential building blocks, metabolites and sig- naling molecules. To reach their site of action AA need to pass through polar epithelial cells. Because AA cannot freely diffuse through the cell membrane, specific AA carriers ensure their transport across the apical and the basolateral membrane. Despite numerous flux studies and huge progress in the iden- tification and characterization of those carrier proteins, AA transport across the basolateral membrane remains not fully understood. The best characterized basolateral transporters in the small intestine and kidney proximal tubule are the obligatory exchanger (antiporter) Lat2-4F2hc (Slc7a8) and y+Lat1- 4F2hc (Slc7a7). Both require the presence of a ”one-way transport” (uniporter) to achieve a net AA efflux. TAT1 (Slc16a10) and LAT4 (Slc43a2) fulfill this requirement with different substrate selectivities: TAT1 facilitates the diffusion of aromatic AA, whereas Lat4 transports branched chain AA, aromatic AA, Met and Pro. The functional cooperation between TAT1 and Lat2-4F2hc has previously been shown using the X. laevis oocyte expression system (Ramadan, Camargo et al. 2007). Furthermore, Lat4 could substitute for TAT1 function. Indeed, TAT1 shows the same localization as LAT2-4F2hc in epithelial cells and is further present in muscle sarcolemma and perivenous hepatocytes. The localization of Lat4 is still unknown, whereas the expression of the two uniporters overlaps only partially. The question addressed in this dissertation is: what is the function of TAT1 and Lat4 in vivo? Using global knock out mouse models we have examined the effect of the absence of the two uniporters on the AA homeostasis and epithelial transport. -
Initiation of Antiviral B Cell Immunity Relies on Innate Signals from Spatially Positioned NKT Cells
Initiation of Antiviral B Cell Immunity Relies on Innate Signals from Spatially Positioned NKT Cells The MIT Faculty has made this article openly available. Please share how this access benefits you. Your story matters. Citation Gaya, Mauro et al. “Initiation of Antiviral B Cell Immunity Relies on Innate Signals from Spatially Positioned NKT Cells.” Cell 172, 3 (January 2018): 517–533 © 2017 The Author(s) As Published http://dx.doi.org/10.1016/j.cell.2017.11.036 Publisher Elsevier Version Final published version Citable link http://hdl.handle.net/1721.1/113555 Terms of Use Creative Commons Attribution 4.0 International License Detailed Terms http://creativecommons.org/licenses/by/4.0/ Article Initiation of Antiviral B Cell Immunity Relies on Innate Signals from Spatially Positioned NKT Cells Graphical Abstract Authors Mauro Gaya, Patricia Barral, Marianne Burbage, ..., Andreas Bruckbauer, Jessica Strid, Facundo D. Batista Correspondence [email protected] (M.G.), [email protected] (F.D.B.) In Brief NKT cells are required for the initial formation of germinal centers and production of effective neutralizing antibody responses against viruses. Highlights d NKT cells promote B cell immunity upon viral infection d NKT cells are primed by lymph-node-resident macrophages d NKT cells produce early IL-4 wave at the follicular borders d Early IL-4 wave is required for efficient seeding of germinal centers Gaya et al., 2018, Cell 172, 517–533 January 25, 2018 ª 2017 The Authors. Published by Elsevier Inc. https://doi.org/10.1016/j.cell.2017.11.036 Article Initiation of Antiviral B Cell Immunity Relies on Innate Signals from Spatially Positioned NKT Cells Mauro Gaya,1,2,* Patricia Barral,2,3 Marianne Burbage,2 Shweta Aggarwal,2 Beatriz Montaner,2 Andrew Warren Navia,1,4,5 Malika Aid,6 Carlson Tsui,2 Paula Maldonado,2 Usha Nair,1 Khader Ghneim,7 Padraic G. -
Structural Studies of Heteromeric Amino Acid Transporters (Hats
Structural studies of Heteromeric Amino acid Transporters (HATs): Validation of the first 3D structural model of a HAT (human 4F2hc/LAT2) and identification of new HAT targets for 3D ‐crystallization Mª Elena Álvarez Marimón ADVERTIMENT . La consulta d’aquesta tesi queda condicionada a l’acceptació de les següents condicions d'ús: La difusió d’aquesta tesi per mitjà del servei TDX ( www.tdx.cat ) i a través del Dipòsit Digital de la UB ( diposit.ub.edu ) ha estat autoritzada pels titulars dels drets de propietat intel·lectual únicament per a usos privats emmarcats en activitats d’investigació i docència. No s’autoritza la seva reproducció amb finalitats de lucre ni la seva difusió i posada a disposició des d’un lloc aliè al servei TDX ni al Dipòsit Digital de la UB. No s’autoritza la presentació del seu contingut en una finestra o marc aliè a TDX o al Dipòsit Digital de la UB (framing). Aquesta reserva de drets afecta tant al resum de presentació de la tesi com als seus continguts. En la utilització o cita de parts de la tesi és obligat indicar el nom de la persona autora. ADVERTENCIA . La consulta de esta tesis queda condicionada a la aceptación de las siguientes condiciones de uso: La difusión de esta tesis por medio del servicio TDR (www.tdx.cat ) y a través del Repositorio Digital de la UB (diposit.ub.edu ) ha sido autorizada por los titulares de los derechos de propiedad intelectual únicamente para usos privados enmarcados en actividades de investigación y docencia. No se autoriza su reproducción con finalidades de lucro ni su difusión y puesta a disposición desde un sitio ajeno al servicio TDR o al Repositorio Digital de la UB. -
Williams Syndrome
Williams syndrome Description Williams syndrome is a developmental disorder that affects many parts of the body. This condition is characterized by mild to moderate intellectual disability or learning problems, unique personality characteristics, distinctive facial features, and heart and blood vessel (cardiovascular) problems. People with Williams syndrome typically have difficulty with visual-spatial tasks such as drawing and assembling puzzles, but they tend to do well on tasks that involve spoken language, music, and learning by repetition (rote memorization). Affected individuals have outgoing, engaging personalities and tend to take an extreme interest in other people. Attention deficit disorder (ADD), problems with anxiety, and phobias are common among people with this disorder. Young children with Williams syndrome have distinctive facial features including a broad forehead, a short nose with a broad tip, full cheeks, and a wide mouth with full lips. Many affected people have dental problems such as teeth that are small, widely spaced, crooked, or missing. In older children and adults, the face appears longer and more gaunt. A form of cardiovascular disease called supravalvular aortic stenosis (SVAS) occurs frequently in people with Williams syndrome. Supravalvular aortic stenosis is a narrowing of the large blood vessel that carries blood from the heart to the rest of the body (the aorta). If this condition is not treated, the aortic narrowing can lead to shortness of breath, chest pain, and heart failure. Other problems with the heart and blood vessels, including high blood pressure (hypertension), have also been reported in people with Williams syndrome. Additional signs and symptoms of Williams syndrome include abnormalities of connective tissue (tissue that supports the body's joints and organs) such as joint problems and soft, loose skin. -
UNIVERSITY of CALIFORNIA, SAN DIEGO Measuring
UNIVERSITY OF CALIFORNIA, SAN DIEGO Measuring and Correlating Blood and Brain Gene Expression Levels: Assays, Inbred Mouse Strain Comparisons, and Applications to Human Disease Assessment A dissertation submitted in partial satisfaction of the requirements for the degree of Doctor of Philosophy in Biomedical Sciences by Mary Elizabeth Winn Committee in charge: Professor Nicholas J Schork, Chair Professor Gene Yeo, Co-Chair Professor Eric Courchesne Professor Ron Kuczenski Professor Sanford Shattil 2011 Copyright Mary Elizabeth Winn, 2011 All rights reserved. 2 The dissertation of Mary Elizabeth Winn is approved, and it is acceptable in quality and form for publication on microfilm and electronically: Co-Chair Chair University of California, San Diego 2011 iii DEDICATION To my parents, Dennis E. Winn II and Ann M. Winn, to my siblings, Jessica A. Winn and Stephen J. Winn, and to all who have supported me throughout this journey. iv TABLE OF CONTENTS Signature Page iii Dedication iv Table of Contents v List of Figures viii List of Tables x Acknowledgements xiii Vita xvi Abstract of Dissertation xix Chapter 1 Introduction and Background 1 INTRODUCTION 2 Translational Genomics, Genome-wide Expression Analysis, and Biomarker Discovery 2 Neuropsychiatric Diseases, Tissue Accessibility and Blood-based Gene Expression 4 Mouse Models of Human Disease 5 Microarray Gene Expression Profiling and Globin Reduction 7 Finding and Accessible Surrogate Tissue for Neural Tissue 9 Genetic Background Effect Analysis 11 SPECIFIC AIMS 12 ENUMERATION OF CHAPTERS -
Structural Basis for Amino Acid Transport by the CAT Family of SLC7 Transporters
ARTICLE DOI: 10.1038/s41467-018-03066-6 OPEN Structural basis for amino acid transport by the CAT family of SLC7 transporters Katharina E.J. Jungnickel 1, Joanne L. Parker1 & Simon Newstead 1 Amino acids play essential roles in cell biology as regulators of metabolic pathways. Arginine in particular is a major signalling molecule inside the cell, being a precursor for both l-ornithine and nitric oxide (NO) synthesis and a key regulator of the mTORC1 pathway. In 1234567890():,; mammals, cellular arginine availability is determined by members of the solute carrier (SLC) 7 family of cationic amino acid transporters. Whereas CAT-1 functions to supply cationic amino acids for cellular metabolism, CAT-2A and -2B are required for macrophage activation and play important roles in regulating inflammation. Here, we present the crystal structure of a close homologue of the mammalian CAT transporters that reveals how these proteins specifically recognise arginine. Our structural and functional data provide a model for cationic amino acid transport in mammalian cells and reveals mechanistic insights into proton- coupled, sodium-independent amino acid transport in the wider APC superfamily. 1 Department of Biochemistry, University of Oxford, South Parks Road, Oxford OX1 3QU, UK. Correspondence and requests for materials should be addressed to S.N. (email: [email protected]) NATURE COMMUNICATIONS | (2018) 9:550 | DOI: 10.1038/s41467-018-03066-6 | www.nature.com/naturecommunications 1 ARTICLE NATURE COMMUNICATIONS | DOI: 10.1038/s41467-018-03066-6 -
Table S1. 103 Ferroptosis-Related Genes Retrieved from the Genecards
Table S1. 103 ferroptosis-related genes retrieved from the GeneCards. Gene Symbol Description Category GPX4 Glutathione Peroxidase 4 Protein Coding AIFM2 Apoptosis Inducing Factor Mitochondria Associated 2 Protein Coding TP53 Tumor Protein P53 Protein Coding ACSL4 Acyl-CoA Synthetase Long Chain Family Member 4 Protein Coding SLC7A11 Solute Carrier Family 7 Member 11 Protein Coding VDAC2 Voltage Dependent Anion Channel 2 Protein Coding VDAC3 Voltage Dependent Anion Channel 3 Protein Coding ATG5 Autophagy Related 5 Protein Coding ATG7 Autophagy Related 7 Protein Coding NCOA4 Nuclear Receptor Coactivator 4 Protein Coding HMOX1 Heme Oxygenase 1 Protein Coding SLC3A2 Solute Carrier Family 3 Member 2 Protein Coding ALOX15 Arachidonate 15-Lipoxygenase Protein Coding BECN1 Beclin 1 Protein Coding PRKAA1 Protein Kinase AMP-Activated Catalytic Subunit Alpha 1 Protein Coding SAT1 Spermidine/Spermine N1-Acetyltransferase 1 Protein Coding NF2 Neurofibromin 2 Protein Coding YAP1 Yes1 Associated Transcriptional Regulator Protein Coding FTH1 Ferritin Heavy Chain 1 Protein Coding TF Transferrin Protein Coding TFRC Transferrin Receptor Protein Coding FTL Ferritin Light Chain Protein Coding CYBB Cytochrome B-245 Beta Chain Protein Coding GSS Glutathione Synthetase Protein Coding CP Ceruloplasmin Protein Coding PRNP Prion Protein Protein Coding SLC11A2 Solute Carrier Family 11 Member 2 Protein Coding SLC40A1 Solute Carrier Family 40 Member 1 Protein Coding STEAP3 STEAP3 Metalloreductase Protein Coding ACSL1 Acyl-CoA Synthetase Long Chain Family Member 1 Protein