Ensemble Modeling of Hepatic Fatty Acid Metabolism with a Synthetic Glyoxylate Shunt
Total Page:16
File Type:pdf, Size:1020Kb
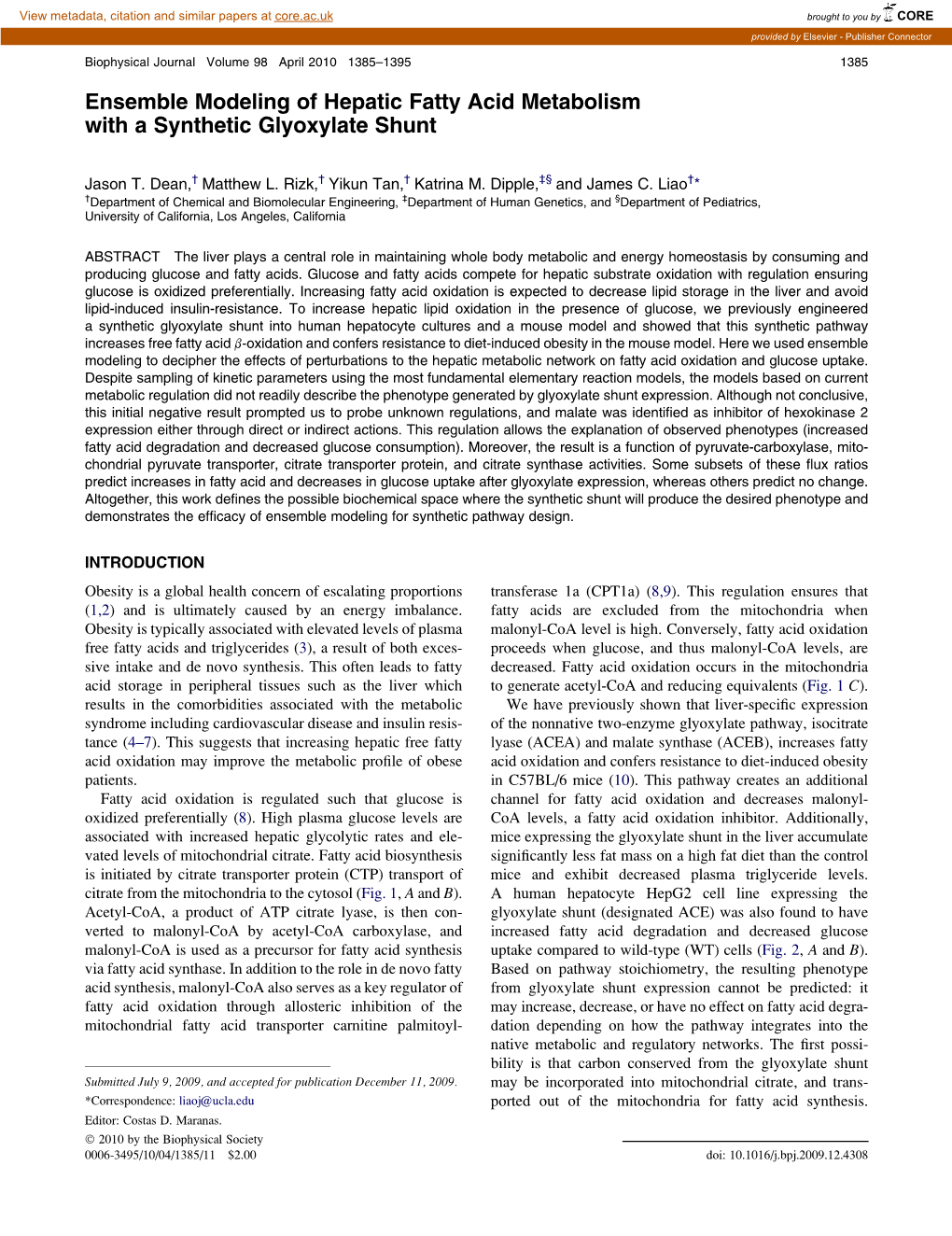
Load more
Recommended publications
-
Generated by SRI International Pathway Tools Version 25.0, Authors S
An online version of this diagram is available at BioCyc.org. Biosynthetic pathways are positioned in the left of the cytoplasm, degradative pathways on the right, and reactions not assigned to any pathway are in the far right of the cytoplasm. Transporters and membrane proteins are shown on the membrane. Periplasmic (where appropriate) and extracellular reactions and proteins may also be shown. Pathways are colored according to their cellular function. Gcf_000238675-HmpCyc: Bacillus smithii 7_3_47FAA Cellular Overview Connections between pathways are omitted for legibility. -
Yeast Genome Gazetteer P35-65
gazetteer Metabolism 35 tRNA modification mitochondrial transport amino-acid metabolism other tRNA-transcription activities vesicular transport (Golgi network, etc.) nitrogen and sulphur metabolism mRNA synthesis peroxisomal transport nucleotide metabolism mRNA processing (splicing) vacuolar transport phosphate metabolism mRNA processing (5’-end, 3’-end processing extracellular transport carbohydrate metabolism and mRNA degradation) cellular import lipid, fatty-acid and sterol metabolism other mRNA-transcription activities other intracellular-transport activities biosynthesis of vitamins, cofactors and RNA transport prosthetic groups other transcription activities Cellular organization and biogenesis 54 ionic homeostasis organization and biogenesis of cell wall and Protein synthesis 48 plasma membrane Energy 40 ribosomal proteins organization and biogenesis of glycolysis translation (initiation,elongation and cytoskeleton gluconeogenesis termination) organization and biogenesis of endoplasmic pentose-phosphate pathway translational control reticulum and Golgi tricarboxylic-acid pathway tRNA synthetases organization and biogenesis of chromosome respiration other protein-synthesis activities structure fermentation mitochondrial organization and biogenesis metabolism of energy reserves (glycogen Protein destination 49 peroxisomal organization and biogenesis and trehalose) protein folding and stabilization endosomal organization and biogenesis other energy-generation activities protein targeting, sorting and translocation vacuolar and lysosomal -
Fatty Acid Biosynthesis
BI/CH 422/622 ANABOLISM OUTLINE: Photosynthesis Carbon Assimilation – Calvin Cycle Carbohydrate Biosynthesis in Animals Gluconeogenesis Glycogen Synthesis Pentose-Phosphate Pathway Regulation of Carbohydrate Metabolism Anaplerotic reactions Biosynthesis of Fatty Acids and Lipids Fatty Acids contrasts Diversification of fatty acids location & transport Eicosanoids Synthesis Prostaglandins and Thromboxane acetyl-CoA carboxylase Triacylglycerides fatty acid synthase ACP priming Membrane lipids 4 steps Glycerophospholipids Control of fatty acid metabolism Sphingolipids Isoprene lipids: Cholesterol ANABOLISM II: Biosynthesis of Fatty Acids & Lipids 1 ANABOLISM II: Biosynthesis of Fatty Acids & Lipids 1. Biosynthesis of fatty acids 2. Regulation of fatty acid degradation and synthesis 3. Assembly of fatty acids into triacylglycerol and phospholipids 4. Metabolism of isoprenes a. Ketone bodies and Isoprene biosynthesis b. Isoprene polymerization i. Cholesterol ii. Steroids & other molecules iii. Regulation iv. Role of cholesterol in human disease ANABOLISM II: Biosynthesis of Fatty Acids & Lipids Lipid Fat Biosynthesis Catabolism Fatty Acid Fatty Acid Degradation Synthesis Ketone body Isoprene Utilization Biosynthesis 2 Catabolism Fatty Acid Biosynthesis Anabolism • Contrast with Sugars – Lipids have have hydro-carbons not carbo-hydrates – more reduced=more energy – Long-term storage vs short-term storage – Lipids are essential for structure in ALL organisms: membrane phospholipids • Catabolism of fatty acids –produces acetyl-CoA –produces reducing -
Letters to Nature
letters to nature Received 7 July; accepted 21 September 1998. 26. Tronrud, D. E. Conjugate-direction minimization: an improved method for the re®nement of macromolecules. Acta Crystallogr. A 48, 912±916 (1992). 1. Dalbey, R. E., Lively, M. O., Bron, S. & van Dijl, J. M. The chemistry and enzymology of the type 1 27. Wolfe, P. B., Wickner, W. & Goodman, J. M. Sequence of the leader peptidase gene of Escherichia coli signal peptidases. Protein Sci. 6, 1129±1138 (1997). and the orientation of leader peptidase in the bacterial envelope. J. Biol. Chem. 258, 12073±12080 2. Kuo, D. W. et al. Escherichia coli leader peptidase: production of an active form lacking a requirement (1983). for detergent and development of peptide substrates. Arch. Biochem. Biophys. 303, 274±280 (1993). 28. Kraulis, P.G. Molscript: a program to produce both detailed and schematic plots of protein structures. 3. Tschantz, W. R. et al. Characterization of a soluble, catalytically active form of Escherichia coli leader J. Appl. Crystallogr. 24, 946±950 (1991). peptidase: requirement of detergent or phospholipid for optimal activity. Biochemistry 34, 3935±3941 29. Nicholls, A., Sharp, K. A. & Honig, B. Protein folding and association: insights from the interfacial and (1995). the thermodynamic properties of hydrocarbons. Proteins Struct. Funct. Genet. 11, 281±296 (1991). 4. Allsop, A. E. et al.inAnti-Infectives, Recent Advances in Chemistry and Structure-Activity Relationships 30. Meritt, E. A. & Bacon, D. J. Raster3D: photorealistic molecular graphics. Methods Enzymol. 277, 505± (eds Bently, P. H. & O'Hanlon, P. J.) 61±72 (R. Soc. Chem., Cambridge, 1997). -
Lipid Deposition and Metabolism in Local and Modern Pig Breeds: a Review
animals Review Lipid Deposition and Metabolism in Local and Modern Pig Breeds: A Review Klavdija Poklukar 1 , Marjeta Candek-Potokarˇ 1,2 , Nina Batorek Lukaˇc 1 , Urška Tomažin 1 and Martin Škrlep 1,* 1 Agricultural Institute of Slovenia, Ljubljana SI-1000, Slovenia; [email protected] (K.P.); [email protected] (M.C.-P.);ˇ [email protected] (N.B.L.); [email protected] (U.T.) 2 University of Maribor, Faculty of Agriculture and Life Sciences, HoˇceSI-2311, Slovenia * Correspondence: [email protected]; Tel.: +386-(0)1-280-52-34 Received: 17 February 2020; Accepted: 29 February 2020; Published: 3 March 2020 Simple Summary: Intensive selective breeding and genetic improvement of relatively few pig breeds led to the abandonment of many low productive local pig breeds. However, local pig breeds are more highly adapted to their specific environmental conditions and feeding resources, and therefore present a valuable genetic resource. They are able to deposit more fat and have a distinct lipogenic capacity, along with a better fatty acid composition than modern breeds. Physiological, biochemical and genetic mechanisms responsible for the differences between fatty and lean breeds are still not fully clarified. The present paper highlights important associations to better understand the underlying mechanisms of lipid deposition in subcutaneous and intramuscular fat between fatty and lean breeds. Abstract: Modern pig breeds, which have been genetically improved to achieve fast growth and a lean meat deposition, differ from local pig breeds with respect to fat deposition, fat specific metabolic characteristics and various other properties. The present review aimed to elucidate the mechanisms underlying the differences between fatty local and modern lean pig breeds in adipose tissue deposition and lipid metabolism, taking into consideration morphological, cellular, biochemical, transcriptomic and proteomic perspectives. -
Activation of Pparα by Fatty Acid Accumulation Enhances Fatty Acid Degradation and Sulfatide Synthesis
Tohoku J. Exp. Med., 2016, 240, 113-122PPARα Activation in Cells due to VLCAD Deficiency 113 Activation of PPARα by Fatty Acid Accumulation Enhances Fatty Acid Degradation and Sulfatide Synthesis * * Yang Yang,1, Yuyao Feng,1, Xiaowei Zhang,2 Takero Nakajima,1 Naoki Tanaka,1 Eiko Sugiyama,3 Yuji Kamijo4 and Toshifumi Aoyama1 1Department of Metabolic Regulation, Shinshu University Graduate School of Medicine, Matsumoto, Nagano, Japan 2Department of Neurosurgery, The Second Hospital of Hebei Medical University, Shijiazhuang, Hebei, China 3Department of Nutritional Science, Nagano Prefectural College, Nagano, Nagano, Japan 4Department of Nephrology, Shinshu University School of Medicine, Matsumoto, Nagano, Japan Very-long-chain acyl-CoA dehydrogenase (VLCAD) catalyzes the first reaction in the mitochondrial fatty acid β-oxidation pathway. VLCAD deficiency is associated with the accumulation of fat in multiple organs and tissues, which results in specific clinical features including cardiomyopathy, cardiomegaly, muscle weakness, and hepatic dysfunction in infants. We speculated that the abnormal fatty acid metabolism in VLCAD-deficient individuals might cause cell necrosis by fatty acid toxicity. The accumulation of fatty acids may activate peroxisome proliferator-activated receptor (PPAR), a master regulator of fatty acid metabolism and a potent nuclear receptor for free fatty acids. We examined six skin fibroblast lines, derived from VLCAD-deficient patients and identified fatty acid accumulation and PPARα activation in these cell lines. We then found that the expression levels of three enzymes involved in fatty acid degradation, including long-chain acyl-CoA synthetase (LACS), were increased in a PPARα-dependent manner. This increased expression of LACS might enhance the fatty acyl-CoA supply to fatty acid degradation and sulfatide synthesis pathways. -
Product Sheet Info
Master Clone List for NR-19279 ® Vibrio cholerae Gateway Clone Set, Recombinant in Escherichia coli, Plates 1-46 Catalog No. NR-19279 Table 1: Vibrio cholerae Gateway® Clones, Plate 1 (NR-19679) Clone ID Well ORF Locus ID Symbol Product Accession Position Length Number 174071 A02 367 VC2271 ribD riboflavin-specific deaminase NP_231902.1 174346 A03 336 VC1877 lpxK tetraacyldisaccharide 4`-kinase NP_231511.1 174354 A04 342 VC0953 holA DNA polymerase III, delta subunit NP_230600.1 174115 A05 388 VC2085 sucC succinyl-CoA synthase, beta subunit NP_231717.1 174310 A06 506 VC2400 murC UDP-N-acetylmuramate--alanine ligase NP_232030.1 174523 A07 132 VC0644 rbfA ribosome-binding factor A NP_230293.2 174632 A08 322 VC0681 ribF riboflavin kinase-FMN adenylyltransferase NP_230330.1 174930 A09 433 VC0720 phoR histidine protein kinase PhoR NP_230369.1 174953 A10 206 VC1178 conserved hypothetical protein NP_230823.1 174976 A11 213 VC2358 hypothetical protein NP_231988.1 174898 A12 369 VC0154 trmA tRNA (uracil-5-)-methyltransferase NP_229811.1 174059 B01 73 VC2098 hypothetical protein NP_231730.1 174075 B02 82 VC0561 rpsP ribosomal protein S16 NP_230212.1 174087 B03 378 VC1843 cydB-1 cytochrome d ubiquinol oxidase, subunit II NP_231477.1 174099 B04 383 VC1798 eha eha protein NP_231433.1 174294 B05 494 VC0763 GTP-binding protein NP_230412.1 174311 B06 314 VC2183 prsA ribose-phosphate pyrophosphokinase NP_231814.1 174603 B07 108 VC0675 thyA thymidylate synthase NP_230324.1 174474 B08 466 VC1297 asnS asparaginyl-tRNA synthetase NP_230942.2 174933 B09 198 -
Fatty Acid Degradation Monounsaturated Fatty Acids
BI/CH 422/622 OUTLINE: OUTLINE: Lipid Degradation (Catabolism) Protein Degradation (Catabolism) FOUR stages in the catabolism of lipids: Digestion Mobilization from tissues (mostly adipose) Inside of cells hormone regulated Protein turnover specific lipases Ubiquitin glycerol Proteosome Activation of fatty acids Amino-Acid Degradation Fatty-acyl CoA Synthetase Transport into mitochondria carnitine Oxidation rationale Saturated FA 4 steps dehydrogenation hydration oxidation thiolase energetics Unsaturated FA Odd-chain FA Ketone Bodies Other organelles Fatty Acid Degradation Monounsaturated Fatty Acids cis trans During first of five ⦚ ⦚ ⦚ ⦚ ⦚ remaining cycles, acyl- CoA dehydrogenase step is skipped, resulting in 1 fewer FADH2. 1 Fatty Acid Degradation Energy from Fatty Acid Catabolism TABLE 17-1a Yield of ATP during Oxidation of One Molecule of PalmitoylOleoyl--CoA to CO2 and H2O Number of NADH or Number of ATP a Enzyme catalyzing the oxidation step FADH2 formed ultimately formed β Oxidation Acyl-CoA dehydrogenase 7 FADH2 10.5 β-Hydroxyacyl-CoA dehydrogenase 8 NADH 20 Citric acid cycle 35 à87.5 ATP Isocitrate dehydrogenase 9 NADH 22.5 α-Ketoglutarate dehydrogenase 9 NADH 22.5 Succinyl-CoA synthetase 16 9b Succinate dehydrogenase à24 ATP 9 FADH2 13.5 Malate dehydrogenase 9 NADH 22.5 Total 120.5 – 2 = 118.5* aThese calculations assume that mitochondrial oxidative phosphorylation produces 1.5 ATP per FADH2 oxidized and 2.5 ATP per NADH oxidized. bGTP produced directly in this step yields ATP in the reaction catalyzed by nucleoside diphosphate kinase (p. 516). *These 2 ”ATP” equivalents were expended in the activation by Fatty acyl–CoA synthetase. Fatty Acid Degradation Oxidation of Polyunsaturated Fatty Acids Results in 1 fewer FADH2 after isomerization, as the acyl-CoA dehydrogenase step is skipped and goes right to the hydratase. -
Investigations of Anaplerosis from Propionyl-Coa
INVESTIGATIONS OF ANAPLEROSIS FROM PROPIONYL-COA PRECURSORS AND FATTY ACID OXIDATION IN THE BRAIN OF VLCAD AND CONTROL MICE by XIAO WANG Submitted in partial fulfillment of the requirements for the Degree of Doctor of Philosophy Thesis Advisor: Henri Brunengraber, M.D., Ph.D. Department of Nutrition CASE WESTERN RESERVE UNIVERISITY May, 2009 CASE WESTERN RESERVE UNIVERSITY SCHOOL OF GRADUATE STUDIES We hereby approve the thesis/dissertation of _____________________________________________________ candidate for the ______________________degree *. (signed)_______________________________________________ (chair of the committee) ________________________________________________ ________________________________________________ ________________________________________________ ________________________________________________ ________________________________________________ (date) _______________________ *We also certify that written approval has been obtained for any proprietary material contained therein. Table of Contents Table of Contents.……………………………………………………………….………i List of Table...……………………………………………………………………………v List of Figures.……………………………………………………………………..….…vi Acknowledgements …………………………………..…………………………..….…xi List of Abbreviations……………………………………………………………………xii Abstract………………………………………………………………………………....xvi CHAPTER 1: Substrate Utilization In The Brain 1.1 Overview of brain energy metabolism..…….………………………………….....1 1.2 The blood-brain barrier ……..……….…….………………………………….…... 2 1.3 Utilization of glucose in the brain………………………………………………..…4 1.3.1 -
Supplementary Informations SI2. Supplementary Table 1
Supplementary Informations SI2. Supplementary Table 1. M9, soil, and rhizosphere media composition. LB in Compound Name Exchange Reaction LB in soil LBin M9 rhizosphere H2O EX_cpd00001_e0 -15 -15 -10 O2 EX_cpd00007_e0 -15 -15 -10 Phosphate EX_cpd00009_e0 -15 -15 -10 CO2 EX_cpd00011_e0 -15 -15 0 Ammonia EX_cpd00013_e0 -7.5 -7.5 -10 L-glutamate EX_cpd00023_e0 0 -0.0283302 0 D-glucose EX_cpd00027_e0 -0.61972444 -0.04098397 0 Mn2 EX_cpd00030_e0 -15 -15 -10 Glycine EX_cpd00033_e0 -0.0068175 -0.00693094 0 Zn2 EX_cpd00034_e0 -15 -15 -10 L-alanine EX_cpd00035_e0 -0.02780553 -0.00823049 0 Succinate EX_cpd00036_e0 -0.0056245 -0.12240603 0 L-lysine EX_cpd00039_e0 0 -10 0 L-aspartate EX_cpd00041_e0 0 -0.03205557 0 Sulfate EX_cpd00048_e0 -15 -15 -10 L-arginine EX_cpd00051_e0 -0.0068175 -0.00948672 0 L-serine EX_cpd00054_e0 0 -0.01004986 0 Cu2+ EX_cpd00058_e0 -15 -15 -10 Ca2+ EX_cpd00063_e0 -15 -100 -10 L-ornithine EX_cpd00064_e0 -0.0068175 -0.00831712 0 H+ EX_cpd00067_e0 -15 -15 -10 L-tyrosine EX_cpd00069_e0 -0.0068175 -0.00233919 0 Sucrose EX_cpd00076_e0 0 -0.02049199 0 L-cysteine EX_cpd00084_e0 -0.0068175 0 0 Cl- EX_cpd00099_e0 -15 -15 -10 Glycerol EX_cpd00100_e0 0 0 -10 Biotin EX_cpd00104_e0 -15 -15 0 D-ribose EX_cpd00105_e0 -0.01862144 0 0 L-leucine EX_cpd00107_e0 -0.03596182 -0.00303228 0 D-galactose EX_cpd00108_e0 -0.25290619 -0.18317325 0 L-histidine EX_cpd00119_e0 -0.0068175 -0.00506825 0 L-proline EX_cpd00129_e0 -0.01102953 0 0 L-malate EX_cpd00130_e0 -0.03649016 -0.79413596 0 D-mannose EX_cpd00138_e0 -0.2540567 -0.05436649 0 Co2 EX_cpd00149_e0 -
Table 4. V. Cholerae Flexgene ORF Collection
Table 4. V. cholerae FLEXGene ORF collection Reference Clone protein PlasmID clone GenBank Locus tag Symbol accession identifier FLEX clone name accession Product name VC0001 NP_062585 VcCD00019918 FLH200476.01F DQ772770 hypothetical protein VC0002 mioC NP_062586 VcCD00019938 FLH200506.01F DQ772771 mioC protein VC0003 thdF NP_062587 VcCD00019958 FLH200531.01F DQ772772 thiophene and furan oxidation protein ThdF VC0004 yidC NP_062588 VcCD00019970 FLH200545.01F DQ772773 inner membrane protein, 60 kDa VC0005 NP_062589 VcCD00061243 FLH236482.01F DQ899316 conserved hypothetical protein VC0006 rnpA NP_062590 VcCD00025697 FLH214799.01F DQ772774 ribonuclease P protein component VC0007 rpmH NP_062591 VcCD00061229 FLH236450.01F DQ899317 ribosomal protein L34 VC0008 NP_062592 VcCD00019917 FLH200475.01F DQ772775 amino acid ABC transporter, ATP-binding protein VC0009 NP_062593 VcCD00019966 FLH200540.01F DQ772776 amino acid ABC transproter, permease protein VC0010 NP_062594 VcCD00019152 FLH199275.01F DQ772777 amino acid ABC transporter, periplasmic amino acid-binding portion VC0011 NP_062595 VcCD00019151 FLH199274.01F DQ772778 hypothetical protein VC0012 dnaA NP_062596 VcCD00017363 FLH174286.01F DQ772779 chromosomal DNA replication initiator DnaA VC0013 dnaN NP_062597 VcCD00017316 FLH174063.01F DQ772780 DNA polymerase III, beta chain VC0014 recF NP_062598 VcCD00019182 FLH199319.01F DQ772781 recF protein VC0015 gyrB NP_062599 VcCD00025458 FLH174642.01F DQ772782 DNA gyrase, subunit B VC0016 NP_229675 VcCD00019198 FLH199346.01F DQ772783 hypothetical protein -
Amino Acid Degradation
BI/CH 422/622 OUTLINE: OUTLINE: Protein Degradation (Catabolism) Digestion Amino-Acid Degradation Inside of cells Protein turnover Dealing with the carbon Ubiquitin Fates of the 29 Activation-E1 Seven Families Conjugation-E2 nitrogen atoms in 20 1. ADENQ Ligation-E3 AA: Proteosome 2. RPH 9 ammonia oxidase Amino-Acid Degradation 18 transamination Ammonia 2 urea one-carbon metabolism free transamination-mechanism to know THF Urea Cycle – dealing with the nitrogen SAM 5 Steps Carbamoyl-phosphate synthetase 3. GSC Ornithine transcarbamylase PLP uses Arginino-succinate synthetase Arginino-succinase 4. MT – one carbon metabolism Arginase 5. FY – oxidase vs oxygenase Energetics Urea Bi-cycle 6. KW – Urea Cycle – dealing with the nitrogen 7. BCAA – VIL Feeding the Urea Cycle Glucose-Alanine Cycle Convergence with Fatty acid-odd chain Free Ammonia Overview Glutamine Glutamate dehydrogenase Overall energetics Amino Acid A. Concepts 1. ConvergentDegradation 2. ketogenic/glucogenic 3. Reactions seen before The SEVEN (7) Families B. Transaminase (A,D,E) / Deaminase (Q,N) Family C. Related to biosynthesis (R,P,H; C,G,S; M,T) 1.Glu Family a. Introduce oxidases/oxygenases b. Introduce one-carbon metabolism (1C) 2.Pyruvate Family a. PLP reactions 3. a-Ketobutyric Family (M,T) a. 1-C metabolism D. Dedicated 1. Aromatic Family (F,Y) a. oxidases/oxygenases 2. a-Ketoadipic Family (K,W) 3. Branched-chain Family (V,I,L) E. Convergence with Fatty Acids: propionyl-CoA 29 N 1 Amino Acid Degradation • Intermediates of the central metabolic pathway • Some amino acids result in more than one intermediate. • Ketogenic amino acids can be converted to ketone bodies.