Austropuccinia Psidii, Causing Myrtle Rust, Has a Gigabase-Sized Genome Shaped by Transposable Elements
Total Page:16
File Type:pdf, Size:1020Kb
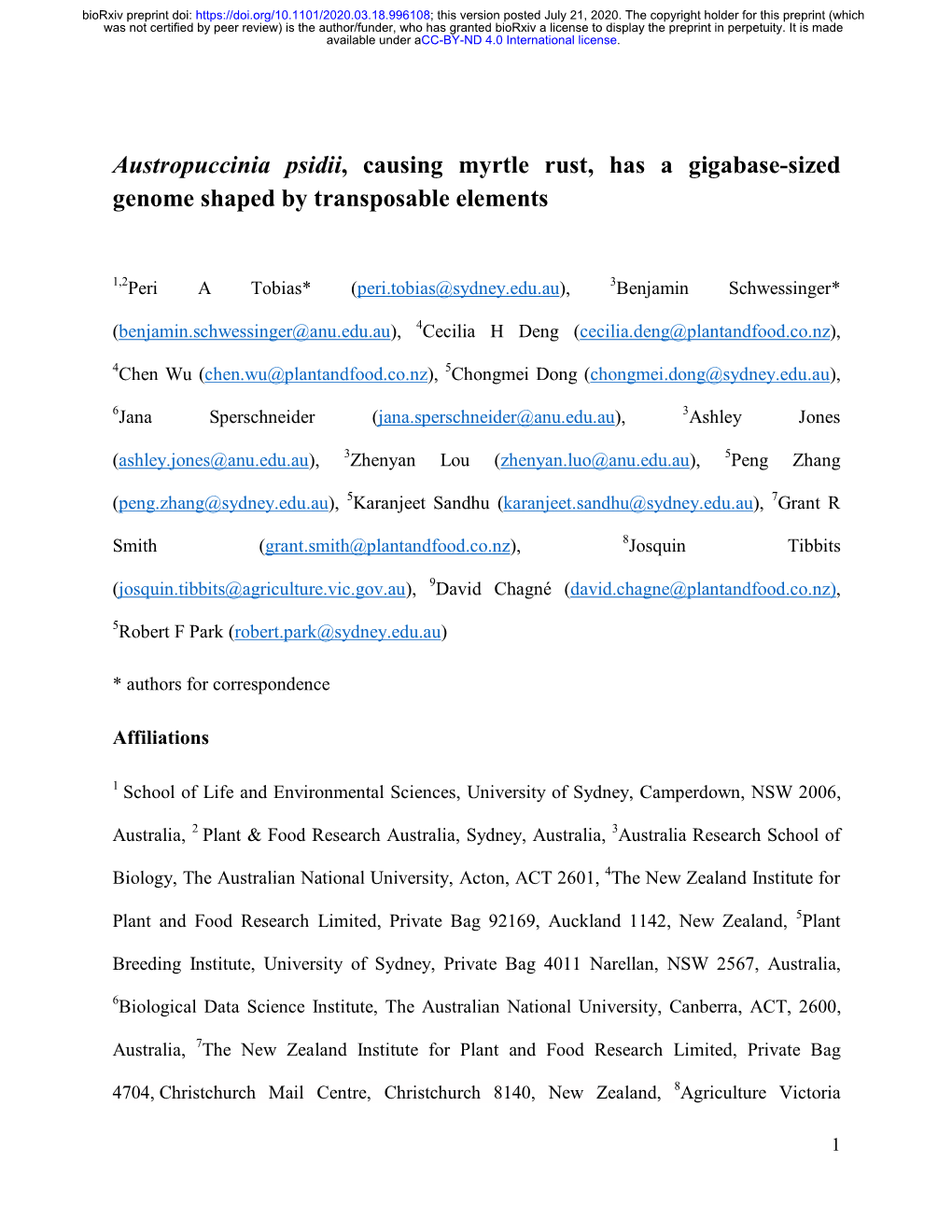
Load more
Recommended publications
-
Bactrocera Tryoni(クインスランドミバエ)に関する 病害虫リスクアナリシス報告書
Bactrocera tryoni(クインスランドミバエ)に関する 病害虫リスクアナリシス報告書 令和3年2月3日 改訂 農林水産省 横浜植物防疫所 主な改訂履歴及び内容 平成 31 年 3 月 25 日 作成 令和 3 年 2 月 3 日 寄主植物の追加(パラミツ等 45 種) 目次 はじめに ..................................................................................................................................................... 1 Ⅰ リスクアナリシス対象の病害虫の生物学的情報(有害動物) ...................................................... 1 1.学名及び分類 .................................................................................................................................. 1 2.地理的分布 ..................................................................................................................................... 1 3.寄主植物及びその日本国内での分布 ............................................................................................ 2 4.寄生部位及びその症状 .................................................................................................................. 2 5.移動分散方法 .................................................................................................................................. 2 6.有害動物の大きさ及び生態 ........................................................................................................... 2 7.媒介性又は被媒介性 ...................................................................................................................... 3 8.被害の程度 ..................................................................................................................................... 3 9.防除 ................................................................................................................................................ -
Jervis Bay Territory Page 1 of 50 21-Jan-11 Species List for NRM Region (Blank), Jervis Bay Territory
Biodiversity Summary for NRM Regions Species List What is the summary for and where does it come from? This list has been produced by the Department of Sustainability, Environment, Water, Population and Communities (SEWPC) for the Natural Resource Management Spatial Information System. The list was produced using the AustralianAustralian Natural Natural Heritage Heritage Assessment Assessment Tool Tool (ANHAT), which analyses data from a range of plant and animal surveys and collections from across Australia to automatically generate a report for each NRM region. Data sources (Appendix 2) include national and state herbaria, museums, state governments, CSIRO, Birds Australia and a range of surveys conducted by or for DEWHA. For each family of plant and animal covered by ANHAT (Appendix 1), this document gives the number of species in the country and how many of them are found in the region. It also identifies species listed as Vulnerable, Critically Endangered, Endangered or Conservation Dependent under the EPBC Act. A biodiversity summary for this region is also available. For more information please see: www.environment.gov.au/heritage/anhat/index.html Limitations • ANHAT currently contains information on the distribution of over 30,000 Australian taxa. This includes all mammals, birds, reptiles, frogs and fish, 137 families of vascular plants (over 15,000 species) and a range of invertebrate groups. Groups notnot yet yet covered covered in inANHAT ANHAT are notnot included included in in the the list. list. • The data used come from authoritative sources, but they are not perfect. All species names have been confirmed as valid species names, but it is not possible to confirm all species locations. -
Brisbane Native Plants by Suburb
INDEX - BRISBANE SUBURBS SPECIES LIST Acacia Ridge. ...........15 Chelmer ...................14 Hamilton. .................10 Mayne. .................25 Pullenvale............... 22 Toowong ....................46 Albion .......................25 Chermside West .11 Hawthorne................. 7 McDowall. ..............6 Torwood .....................47 Alderley ....................45 Clayfield ..................14 Heathwood.... 34. Meeandah.............. 2 Queensport ............32 Trinder Park ...............32 Algester.................... 15 Coopers Plains........32 Hemmant. .................32 Merthyr .................7 Annerley ...................32 Coorparoo ................3 Hendra. .................10 Middle Park .........19 Rainworth. ..............47 Underwood. ................41 Anstead ....................17 Corinda. ..................14 Herston ....................5 Milton ...................46 Ransome. ................32 Upper Brookfield .......23 Archerfield ...............32 Highgate Hill. ........43 Mitchelton ...........45 Red Hill.................... 43 Upper Mt gravatt. .......15 Ascot. .......................36 Darra .......................33 Hill End ..................45 Moggill. .................20 Richlands ................34 Ashgrove. ................26 Deagon ....................2 Holland Park........... 3 Moorooka. ............32 River Hills................ 19 Virginia ........................31 Aspley ......................31 Doboy ......................2 Morningside. .........3 Robertson ................42 Auchenflower -
Syzygium and Related Genera (Myrtaceae) in Auckland
12 SYZYGIUM AND RELATED GENERA (MYRTACEAE) IN AUCKLAND R.O. Gardner The Australian members of this alliance have been expertly revised by Hyland (1983) making it possible to improve acquaintance with the five species in Syzygium Acmena and Waterhousea that are grown in Auckland for ornament and shelter. These are essentially trees of warm latitudes along Australias eastern coast but they find our climate and probably richer soils congenial and often it seems grow better here than in their homeland. None however have properly naturalized though undispersed juveniles and a few adults do occur. The disposition into genera is based largely upon characters of the. fruit and seed. What appears to be a simple baccate "monkey apple" may conceal unusual features like ruminate cotyledons or a missing seed coat so the fruits of these species produced here in abundance are very interesting to dissect and compare. Leaf silhouettes of the five Australian species are shown in Figure IA. Acmena smithii lillipilli monkey apple (NZ) Very common around Auckland as a street or specimen tree and in hedges. Seedlings occur close to the plantings but most succumb to scale and thrips. Some of the seedlings at Purewa cemetery have a lignotuber unlike most Australian forms of the species (Figure IB). Waterhousea floribunda weeping lillipilli (formerly Syzygium floribundum Eugenia ventenatii) Only seen in a few old gardens e.g. at Highwic The Pines Western Park being fine trees to c. 15 m tall 80 cm dbh and especially beautiful in spring with their pendent new foliage of pink and yellow; a species which should be much more often grown in this country. -
Post-Fire Recovery of Woody Plants in the New England Tableland Bioregion
Post-fire recovery of woody plants in the New England Tableland Bioregion Peter J. ClarkeA, Kirsten J. E. Knox, Monica L. Campbell and Lachlan M. Copeland Botany, School of Environmental and Rural Sciences, University of New England, Armidale, NSW 2351, AUSTRALIA. ACorresponding author; email: [email protected] Abstract: The resprouting response of plant species to fire is a key life history trait that has profound effects on post-fire population dynamics and community composition. This study documents the post-fire response (resprouting and maturation times) of woody species in six contrasting formations in the New England Tableland Bioregion of eastern Australia. Rainforest had the highest proportion of resprouting woody taxa and rocky outcrops had the lowest. Surprisingly, no significant difference in the median maturation length was found among habitats, but the communities varied in the range of maturation times. Within these communities, seedlings of species killed by fire, mature faster than seedlings of species that resprout. The slowest maturing species were those that have canopy held seed banks and were killed by fire, and these were used as indicator species to examine fire immaturity risk. Finally, we examine whether current fire management immaturity thresholds appear to be appropriate for these communities and find they need to be amended. Cunninghamia (2009) 11(2): 221–239 Introduction Maturation times of new recruits for those plants killed by fire is also a critical biological variable in the context of fire Fire is a pervasive ecological factor that influences the regimes because this time sets the lower limit for fire intervals evolution, distribution and abundance of woody plants that can cause local population decline or extirpation (Keith (Whelan 1995; Bond & van Wilgen 1996; Bradstock et al. -
Biodiversity Summary: Port Phillip and Westernport, Victoria
Biodiversity Summary for NRM Regions Species List What is the summary for and where does it come from? This list has been produced by the Department of Sustainability, Environment, Water, Population and Communities (SEWPC) for the Natural Resource Management Spatial Information System. The list was produced using the AustralianAustralian Natural Natural Heritage Heritage Assessment Assessment Tool Tool (ANHAT), which analyses data from a range of plant and animal surveys and collections from across Australia to automatically generate a report for each NRM region. Data sources (Appendix 2) include national and state herbaria, museums, state governments, CSIRO, Birds Australia and a range of surveys conducted by or for DEWHA. For each family of plant and animal covered by ANHAT (Appendix 1), this document gives the number of species in the country and how many of them are found in the region. It also identifies species listed as Vulnerable, Critically Endangered, Endangered or Conservation Dependent under the EPBC Act. A biodiversity summary for this region is also available. For more information please see: www.environment.gov.au/heritage/anhat/index.html Limitations • ANHAT currently contains information on the distribution of over 30,000 Australian taxa. This includes all mammals, birds, reptiles, frogs and fish, 137 families of vascular plants (over 15,000 species) and a range of invertebrate groups. Groups notnot yet yet covered covered in inANHAT ANHAT are notnot included included in in the the list. list. • The data used come from authoritative sources, but they are not perfect. All species names have been confirmed as valid species names, but it is not possible to confirm all species locations. -
Blair's Rainforest Inventory
Enoggera creek (Herston/Wilston) rainforest inventory Prepared by Blair Bartholomew 28-Jan-02 Botanical Name Common Name: tree, shrub, Derivation (Pronunciation) vine, timber 1. Acacia aulacocarpa Brown salwood, hickory/brush Acacia from Greek ”akakia (A), hê”, the shittah tree, Acacia arabica; (changed to Acacia ironbark/broad-leaved/black/grey which is derived from the Greek “akanth-a [a^k], ês, hê, (akê A)” a thorn disparrima ) wattle, gugarkill or prickle (alluding to the spines on the many African and Asian species first described); aulacocarpa from Greek “aulac” furrow and “karpos” a fruit, referring to the characteristic thickened transverse bands on the a-KAY-she-a pod. Disparrima from Latin “disparrima”, the most unlike, dissimilar, different or unequal referring to the species exhibiting the greatest difference from other renamed species previously described as A aulacocarpa. 2. Acacia melanoxylon Black wood/acacia/sally, light Melanoxylon from Greek “mela_s” black or dark: and “xulon” wood, cut wood, hickory, silver/sally/black- and ready for use, or tree, referring to the dark timber of this species. hearted wattle, mudgerabah, mootchong, Australian blackwood, native ash, bastard myall 3. Acmena hemilampra Broad-leaved lillypilly, blush satin Acmena from Greek “Acmenae” the nymphs of Venus who were very ash, water gum, cassowary gum beautiful, referring to the attractive flowers and fruits. A second source says that Acmena was a nymph dedicated to Venus. This derivation ac-ME-na seems the most likely. Finally another source says that the name is derived from the Latin “Acmena” one of the names of the goddess Venus. Hemilampra from Greek “hemi” half and “lampro”, bright, lustrous or shining, referring to the glossy upper leaf surface. -
Myrtle Rust Reviewed the Impacts of the Invasive Plant Pathogen Austropuccinia Psidii on the Australian Environment R
Myrtle Rust reviewed The impacts of the invasive plant pathogen Austropuccinia psidii on the Australian environment R. O. Makinson 2018 DRAFT CRCPLANTbiosecurity CRCPLANTbiosecurity © Plant Biosecurity Cooperative Research Centre, 2018 ‘Myrtle Rust reviewed: the impacts of the invasive pathogen Austropuccinia psidii on the Australian environment’ is licenced by the Plant Biosecurity Cooperative Research Centre for use under a Creative Commons Attribution 4.0 Australia licence. For licence conditions see: https://creativecommons.org/licenses/by/4.0/ This Review provides background for the public consultation document ‘Myrtle Rust in Australia – a draft Action Plan’ available at www.apbsf.org.au Author contact details R.O. Makinson1,2 [email protected] 1Bob Makinson Consulting ABN 67 656 298 911 2The Australian Network for Plant Conservation Inc. Cite this publication as: Makinson RO (2018) Myrtle Rust reviewed: the impacts of the invasive pathogen Austropuccinia psidii on the Australian environment. Plant Biosecurity Cooperative Research Centre, Canberra. Front cover: Top: Spotted Gum (Corymbia maculata) infected with Myrtle Rust in glasshouse screening program, Geoff Pegg. Bottom: Melaleuca quinquenervia infected with Myrtle Rust, north-east NSW, Peter Entwistle This project was jointly funded through the Plant Biosecurity Cooperative Research Centre and the Australian Government’s National Environmental Science Program. The Plant Biosecurity CRC is established and supported under the Australian Government Cooperative Research Centres Program. EXECUTIVE SUMMARY This review of the environmental impacts of Myrtle Rust in Australia is accompanied by an adjunct document, Myrtle Rust in Australia – a draft Action Plan. The Action Plan was developed in 2018 in consultation with experts, stakeholders and the public. The intent of the draft Action Plan is to provide a guiding framework for a specifically environmental dimension to Australia’s response to Myrtle Rust – that is, the conservation of native biodiversity at risk. -
Ecology of Pyrmont Peninsula 1788 - 2008
Transformations: Ecology of Pyrmont peninsula 1788 - 2008 John Broadbent Transformations: Ecology of Pyrmont peninsula 1788 - 2008 John Broadbent Sydney, 2010. Ecology of Pyrmont peninsula iii Executive summary City Council’s ‘Sustainable Sydney 2030’ initiative ‘is a vision for the sustainable development of the City for the next 20 years and beyond’. It has a largely anthropocentric basis, that is ‘viewing and interpreting everything in terms of human experience and values’(Macquarie Dictionary, 2005). The perspective taken here is that Council’s initiative, vital though it is, should be underpinned by an ecocentric ethic to succeed. This latter was defined by Aldo Leopold in 1949, 60 years ago, as ‘a philosophy that recognizes[sic] that the ecosphere, rather than any individual organism[notably humans] is the source and support of all life and as such advises a holistic and eco-centric approach to government, industry, and individual’(http://dictionary.babylon.com). Some relevant considerations are set out in Part 1: General Introduction. In this report, Pyrmont peninsula - that is the communities of Pyrmont and Ultimo – is considered as a microcosm of the City of Sydney, indeed of urban areas globally. An extensive series of early views of the peninsula are presented to help the reader better visualise this place as it was early in European settlement (Part 2: Early views of Pyrmont peninsula). The physical geography of Pyrmont peninsula has been transformed since European settlement, and Part 3: Physical geography of Pyrmont peninsula describes the geology, soils, topography, shoreline and drainage as they would most likely have appeared to the first Europeans to set foot there. -
I Is the Sunda-Sahul Floristic Exchange Ongoing?
Is the Sunda-Sahul floristic exchange ongoing? A study of distributions, functional traits, climate and landscape genomics to investigate the invasion in Australian rainforests By Jia-Yee Samantha Yap Bachelor of Biotechnology Hons. A thesis submitted for the degree of Doctor of Philosophy at The University of Queensland in 2018 Queensland Alliance for Agriculture and Food Innovation i Abstract Australian rainforests are of mixed biogeographical histories, resulting from the collision between Sahul (Australia) and Sunda shelves that led to extensive immigration of rainforest lineages with Sunda ancestry to Australia. Although comprehensive fossil records and molecular phylogenies distinguish between the Sunda and Sahul floristic elements, species distributions, functional traits or landscape dynamics have not been used to distinguish between the two elements in the Australian rainforest flora. The overall aim of this study was to investigate both Sunda and Sahul components in the Australian rainforest flora by (1) exploring their continental-wide distributional patterns and observing how functional characteristics and environmental preferences determine these patterns, (2) investigating continental-wide genomic diversities and distances of multiple species and measuring local species accumulation rates across multiple sites to observe whether past biotic exchange left detectable and consistent patterns in the rainforest flora, (3) coupling genomic data and species distribution models of lineages of known Sunda and Sahul ancestry to examine landscape-level dynamics and habitat preferences to relate to the impact of historical processes. First, the continental distributions of rainforest woody representatives that could be ascribed to Sahul (795 species) and Sunda origins (604 species) and their dispersal and persistence characteristics and key functional characteristics (leaf size, fruit size, wood density and maximum height at maturity) of were compared. -
Islander Perceptions of Invasive Alien Species: the Role of Socio- Economy and Culture in Small Isolated Islands of French Polynesia (South Pacific)
J.-Y. Meyer and M. Fourdrigniez Meyer, J.-Y. and M. Fourdrigniez. Islander perceptions of invasive alien species: the role of socio- economy and culture in small isolated islands of French Polynesia (South Pacific) Islander perceptions of invasive alien species: the role of socio- economy and culture in small isolated islands of French Polynesia (South Pacific) J.-Y. Meyer1 and M. Fourdrigniez2 1Délégation à la Recherche, Government of French Polynesia, B.P. 20981, 98713 Papeete, Tahiti, French Polynesia. <[email protected]>. 2Groupement Espèces Envahissantes, Bioconsulting, B.P. 50902, 98716 Pirae, Tahiti, French Polynesia. Abstract Islands, often celebrated as natural laboratories for evolution and ecology, also provide unique experimental grounds for societal studies. Although biological invasions are widely recognised as one of the main causes of biodiversity erosion and a driver of global change, the human perception of invasive species may vary at regional and local levels, especially in societies with different levels of socio-economic development and cultures. This study was conducted in French Polynesia (South Pacific), a territory formed by 120 tropical and subtropical oceanic islands (76 being inhabited) divided into five archipelagos (Austral, Marquesas, Society, Tuamotu, and Gambier Is), comprising both highly populated and urbanised islands (such as Tahiti in the Society Is) and less populated and very small islands, sometimes very isolated (without airstrips) and where traditional life style and strong dependence on natural resources still persist. During an eight-month education and prevention campaign targeting alien plant and animal species legally declared invasive in French Polynesia, public meetings were organised on 19 small islands for a total of 2,045 consulted people in 41 different villages. -
Jane Gibbs BA Gdiped Gdippsych
Asthma and Plants: Chemotaxonomic Relationships and Patterns of Asthma Incidence and Respiratory Symptoms, in Urban Coastal Versus Rural Highland Areas in South-East Queensland, Australia, with Special Reference to the Family Myrtaceae Author Gibbs, Jane Published 2007 Thesis Type Thesis (PhD Doctorate) School School of Public Health DOI https://doi.org/10.25904/1912/3128 Copyright Statement The author owns the copyright in this thesis, unless stated otherwise. Downloaded from http://hdl.handle.net/10072/366726 Griffith Research Online https://research-repository.griffith.edu.au ASTHMA AND PLANTS: CHEMOTAXONOMIC RELATIONSHIPS AND PATTERNS OF ASTHMA INCIDENCE AND RESPIRATORY SYMPTOMS, IN URBAN COASTAL VERSUS RURAL HIGHLAND AREAS IN SOUTH-EAST QUEENSLAND, AUSTRALIA, WITH SPECIAL REFERENCE TO THE FAMILY MYRTACEAE. Jane Gibbs BA GDipEd GDipPsych School of Public Health Faculty of Health Science Griffith University Submitted in fulfilment of the requirements of the degree of Doctor of Philosophy on 22 December 2006 ii STATEMENT OF ORIGINALITY This work has not been previously submitted for a degree or diploma in a university. To the best of my knowledge and belief, this thesis contains no material previously published or written by another person except where due reference is made in the thesis itself. Jane Gibbs iii Dedication To my daughter Anna, and all the children who live with asthma, for providing the reason to begin this task, and to her grandmother, and my mother, Marie Gibbs (1926-1995), for providing the persistence required to finish it. iv Abstract This thesis represents an exploratory and iterative study into the relationships of Australian native plants from the family Myrtaceae, with respiratory symptoms, specifically asthma.