Thermal Properties of Graphene and Nanostructured Carbon Materials Alexander A
Total Page:16
File Type:pdf, Size:1020Kb
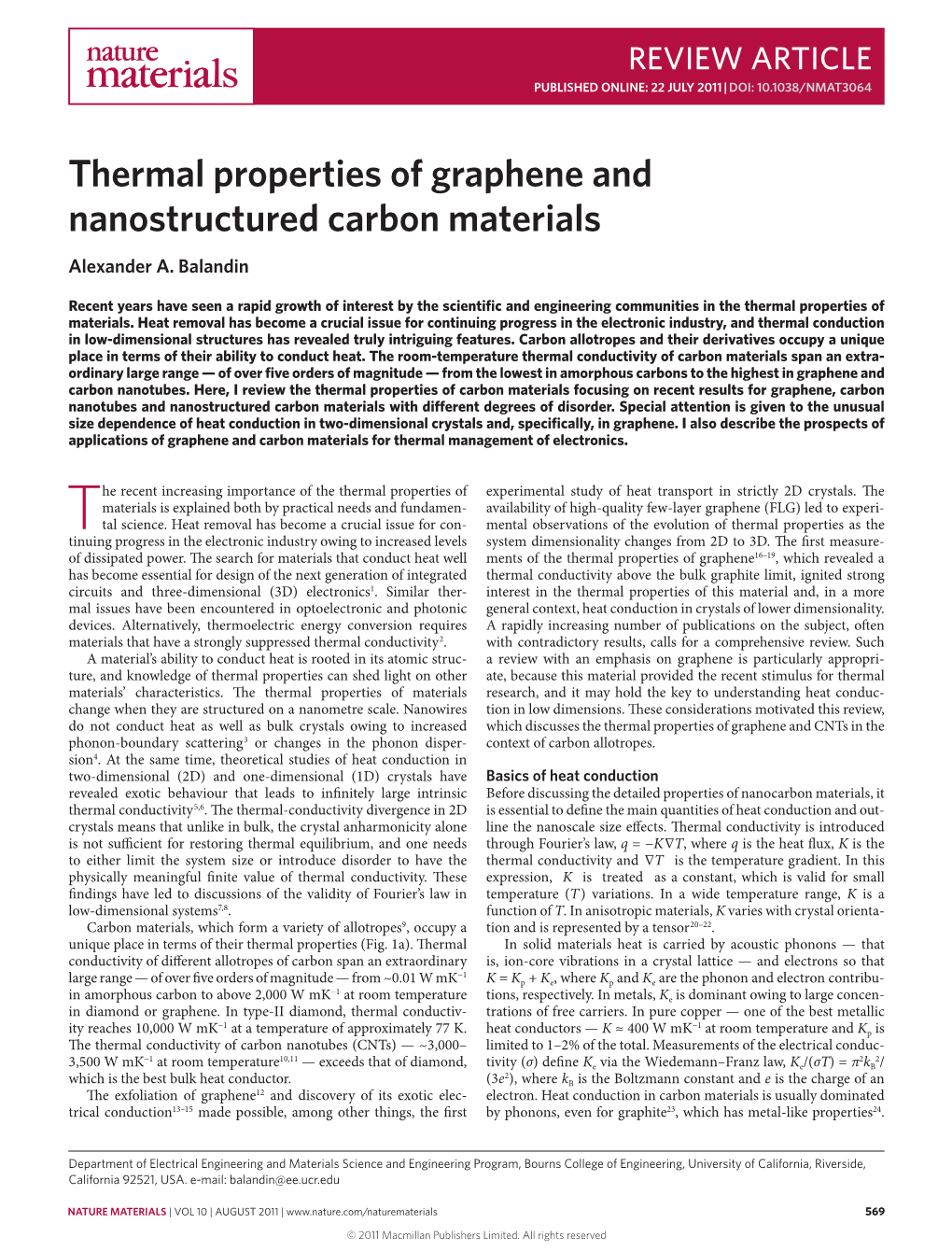
Load more
Recommended publications
-
The Era of Carbon Allotropes Andreas Hirsch
commentary The era of carbon allotropes Andreas Hirsch Twenty-five years on from the discovery of 60C , the outstanding properties and potential applications of the synthetic carbon allotropes — fullerenes, nanotubes and graphene — overwhelmingly illustrate their unique scientific and technological importance. arbon is the element in the periodic consist of extended networks of sp3- and 1985, with the advent of fullerenes (Fig. 1), table that provides the basis for life sp2 -hybridized carbon atoms, respectively. which were observed for the first time by Con Earth. It is also important for Both forms show unique physical properties Kroto et al.3. This serendipitous discovery many technological applications, ranging such as hardness, thermal conductivity, marked the beginning of an era of synthetic from drugs to synthetic materials. This lubrication behaviour or electrical carbon allotropes. Now, as we celebrate role is a consequence of carbon’s ability conductivity. Conceptually, many other buckminsterfullerene’s 25th birthday, it is to bind to itself and to nearly all elements ways to construct carbon allotropes are also the time to reflect on a growing family in almost limitless variety. The resulting possible by altering the periodic binding of synthetic carbon allotropes, which structural diversity of organic compounds motif in networks consisting of sp3-, sp2- includes the synthesis of carbon nanotubes and molecules is accompanied by a broad and sp-hybridized carbon atoms1,2. As a in 19914 and the rediscovery of graphene range of -
Carbon Nanostructures – from Molecules to Functionalised Materials
Digital Comprehensive Summaries of Uppsala Dissertations from the Faculty of Science and Technology 1537 Carbon Nanostructures – from Molecules to Functionalised Materials Fullerene-Ferrocene Oligomers, Graphene Modification and Deposition MICHAEL NORDLUND ACTA UNIVERSITATIS UPSALIENSIS ISSN 1651-6214 ISBN 978-91-513-0019-1 UPPSALA urn:nbn:se:uu:diva-327189 2017 Dissertation presented at Uppsala University to be publicly examined in A1:107a, BMC, Husargatan 3, Uppsala, Friday, 22 September 2017 at 09:15 for the degree of Doctor of Philosophy. The examination will be conducted in English. Faculty examiner: Professor Mogens Brøndsted Nielsen (Copenhagen University, Department of chemistry). Abstract Nordlund, M. 2017. Carbon Nanostructures – from Molecules to Functionalised Materials. Fullerene-Ferrocene Oligomers, Graphene Modification and Deposition. Digital Comprehensive Summaries of Uppsala Dissertations from the Faculty of Science and Technology 1537. 64 pp. Uppsala: Acta Universitatis Upsaliensis. ISBN 978-91-513-0019-1. The work described in this thesis concerns development, synthesis and characterisation of new molecular compounds and materials based on the carbon allotropes fullerene (C60) and graphene. A stepwise strategy to a symmetric ferrocene-linked dumbbell of fulleropyrrolidines was developed. The versatility of this approach was demonstrated in the synthesis of a non- symmetric fulleropyrrolidine-ferrocene-tryptophan triad. A new tethered bis-aldehyde, capable of regiospecific bis-pyrrolidination of a C60-fullerene in predominantly trans fashion, was designed, synthesised and reacted with glycine and C60 to yield the desired N-unfunctionalised bis(pyrrolidine)fullerene. A catenane dimer composed of two bis(pyrrolidine)fullerenes was obtained as a minor co-product. From the synthesis of the N-methyl analogue, the catenane dimer could be separated from the monomeric main product and fully characterised by NMR spectroscopy. -
Graphene: a Peculiar Allotrope of Carbon
Graphene: A Peculiar Allotrope Of Carbon Laxmi Nath Bhattarai Department of Physics, Butwal Multiple Campus Correspondence: [email protected] Abstract Graphene is a two dimensional one atom thick allotrope of Carbon. Electrons in grapheme behave as massless relativistic particles. It is a 2 dimensional nanomaterial with many peculiar properties. In grapheme both integral and fractional quantum Hall effects are observed. Many practical application are seen from use of Graphene material. Keywords: Graphene, allotrope, Quantum Hall effect, nanomaterial. Introduction Graphene is a two dimensional allotropic form of Honey comb structure of Graphene carbon. This was discovered in 2004. Diamond and Graphene is considered as the mother of all graphitic Graphite are three dimensional allotropes of carbon materials because it is the building block of carbon which are known from ancient time. One dimensional materials of all other dimensions (Srinivasan, 2007). carbon nanotubes were discovered in 1991 and Griphite is obtained by the stacking of Graphene zero dimensional Fullerenes were discovered in layers, Diamond can be obtained from Graphene under 1985. Graphene was experimentally extracted from extreme pressure and temperatures. One-dimensional 3-dimensional Graphite by Physisists Andre Geim and carbon nanotubes can be obtained by rolling and Konstantin Novoselov of Manchester University UK Zero–dimensional Fullerenes is obtained by wrapping in 2004. For their remarkable work they were awarded Graphene layers. the Nobel Prize of Physics for the year 2010. Properties Structure The young material Graphene is found to have Graphene is a mono layer of Carbon atoms packed following unique properties: into a honeycomb crystal structure. Graphene sheets 2 are one atom thick 2-dimensional layers of sp – bonded a) It is the thinnest material in the universe and the carbon. -
Carbon-Based Nanomaterials/Allotropes: a Glimpse of Their Synthesis, Properties and Some Applications
materials Review Carbon-Based Nanomaterials/Allotropes: A Glimpse of Their Synthesis, Properties and Some Applications Salisu Nasir 1,2,* ID , Mohd Zobir Hussein 1,* ID , Zulkarnain Zainal 3 and Nor Azah Yusof 3 1 Materials Synthesis and Characterization Laboratory (MSCL), Institute of Advanced Technology (ITMA), Universiti Putra Malaysia, 43400 Serdang, Selangor, Malaysia 2 Department of Chemistry, Faculty of Science, Federal University Dutse, 7156 Dutse, Jigawa State, Nigeria 3 Department of Chemistry, Faculty of Science, Universiti Putra Malaysia, 43400 Serdang, Selangor, Malaysia; [email protected] (Z.Z.); [email protected] (N.A.Y.) * Correspondence: [email protected] (S.N.); [email protected] (M.Z.H.); Tel.: +60-1-2343-3858 (M.Z.H.) Received: 19 November 2017; Accepted: 3 January 2018; Published: 13 February 2018 Abstract: Carbon in its single entity and various forms has been used in technology and human life for many centuries. Since prehistoric times, carbon-based materials such as graphite, charcoal and carbon black have been used as writing and drawing materials. In the past two and a half decades or so, conjugated carbon nanomaterials, especially carbon nanotubes, fullerenes, activated carbon and graphite have been used as energy materials due to their exclusive properties. Due to their outstanding chemical, mechanical, electrical and thermal properties, carbon nanostructures have recently found application in many diverse areas; including drug delivery, electronics, composite materials, sensors, field emission devices, energy storage and conversion, etc. Following the global energy outlook, it is forecasted that the world energy demand will double by 2050. This calls for a new and efficient means to double the energy supply in order to meet the challenges that forge ahead. -
2. Allotropy in Carbon the Property Due to Which an Element Exists In
SUBJECT-CHEMISTRY DATE- 22/11/2020 CLASS- X TOPICS- Chap. 4 Carbon and its Compounds Carbon is one of the most essential components of living organisms. There are two stable isotopes of carbon C-12 and C-13. After these two one more isotope of carbon is present C-14. Carbon is used for radiocarbon dating One of the most amazing properties of carbon is its ability to make long carbon chains and rings. This property of carbon is known as catenation. Carbon has many special abilities out of all one unique ability is that carbon forms double or triple bonds with itself and with other electronegative atoms like oxygen and nitrogen. These two properties of carbon i.e catenation and multiple bond formation, it has the number of allotropic forms. Allotrope is nothing but the existence of an element in many forms which will have different physical property but will have similar chemical properties and its forms are called allotropes of allotropic forms. Allotropes are defined as the two or more physical forms of one element. These allotropes are all based on carbon atoms but exhibit different physical properties, especially with regard to hardness. The two common, crystalline allotropes of carbon are diamond and graphite. Carbon shows allotropy because it exists in different forms of carbon. Though these allotropes of carbon have a different crystal structure and different physical properties, their chemical properties are the same and show similar chemical properties. Both diamond and graphite have symbol C. Both give off carbon dioxide when strongly heated in the presence of oxygen. -
Catching up with Carbon Japan Fights to Stay on Top of a Field It Pioneered
NANOCARBON IN JAPAN SPOTLIGHT Catching up with carbon Japan fights to stay on top of a field it pioneered. BY TIM HORNYAK n September last year, a handful of Nagoya University. “It was the most exciting the stability and strength to build complex researchers were sitting around a com- moment I ever had in my life.” molecules — into new materials with useful puter monitor in chemist Kenchiro Itami’s Itami thinks he has good reason to be so properties, and it has built a strong industrial Ilab at Japan’s Nagoya University as one loaded excited. “The discovery of a new form of car- sector from those basic research efforts. a file showing the results of an X-ray crystal- bon has always opened up new science and But in the past decade or so, Japan has found lography scan. Within seconds, the room technology — fullerenes are a great example,” itself beset by international competition, as erupted: scientists were on their feet, cheering he says, referring to the all-carbon molecular progress in the field has shifted abroad. Now, and exchanging high fives. In front of them spheres created by scientists at Rice University Japanese researchers are fighting to maintain was a 3D representation of a carbon nanobelt in Houston, Texas, in 1985. their global prominence. — a new molecule of carbon that the team Chemists have indeed tried many things had successfully synthesized after 12 years of when it comes to constructing exotic forms, NO SMALL PEDIGREE ILLUSTRATION BY PETER HORVATH; PHOTOS: GETTY PHOTOS: HORVATH; PETER BY ILLUSTRATION painstaking effort. -
Allotropes of Carbon Nanotubes with Mwcnts Kevin James Hughes* Professor, Department of Engineering, Simpson College, North C Street Indianola, Iowa, USA
dicine e & N om a n n a o t N e f c o h Hughes. J Nanomed Nanotechnol 2015, 6:4 l n Journal of a o n l o r g u DOI: 10.4172/2157-7439.1000302 y o J ISSN: 2157-7439 Nanomedicine & Nanotechnology Short Communication Open Access Allotropes of Carbon Nanotubes with MWCNTs Kevin James Hughes* Professor, Department of Engineering, Simpson College, North C Street Indianola, Iowa, USA Abstract Carbon nanotubes (CNTs) are allotropes of carbon with a nanostructure which will have a length-to-diameter quantitative relation bigger than 1,000,000. Techniques are developed to supply nanotubes in sizeable quantities, together with arc discharge, optical device ablation, and chemical vapor deposition. Developments within the past few years have illustrated the possibly revolutionizing impact of nanomaterials, particularly in medical specialty imaging, drug delivery, biosensing, and also the style of practical nanocomposites. Strategies to effectively interface proteins with nanomaterials for realizing these applications still evolve. The high surface-to-volume quantitative relation offered by nanoparticles resulted within the concentration of the immobilized entity being significantly beyond that afforded by different materials. There has conjointly been AN increasing interest in understanding the influence of nanomaterials on the structure and performance of proteins. varied immobilization strategies are developed, and particularly, specific attachment of enzymes on carbon nanotubes has been a crucial focus of attention. With the growing attention paid to cascade catalyst reaction, it’s attainable that multienzyme coimmobilization would be one among ensuing goals within the future. During this paper, we tend to concentrate on advances in methodology for catalyst immobilization on carbon nanotubes. -
Stronger Than Steel: Carbon Nanotubes
StrongerStronger thanthan Steel:Steel: CarbonCarbon NanotubesNanotubes Timothy Gay Jessica Kaufman Marc McGuigan Boston University December 15, 2005 HowHow smallsmall isis nanonanonano??? Nano Nano Nano-hype! - - hype! hype! TodayTodayToday’s’’ss NanotubeNanotube WorkshopWorkshop • The Chemistry of Carbon A New Form of Carbon: Nanotubes Activity: Building Nanotube Models • Nanotubes in Biology and Medicine Biosensors Filtration of Biocontaminants • Carbon Nanotubes – Stronger than steel Tensile strength of Carbon Nanotubes Composite Materials Applications ChemistryChemistry ofof CarbonCarbon FormsForms ofof CarbonCarbon • Carbon was discovered in prehistory and was known to the ancients •The allotropes of carbon are the different molecular configurations that pure carbon can take. •The three relatively well-known allotropes of carbon are amorphous carbon, graphite and diamond. • Several exotic allotropes have also been synthesized or discovered, including fullerenes and carbon nanotubes. GraphiteGraphite • Graphite is a conductor, and can be used as the material in the electrodes of an electrical arc lamp, or as the lead in pencils. • Each carbon atom is covalently bonded to three other surrounding carbon atoms. •Each carbon atom possesses an sp2 orbital hybridization. The pi orbital electrons delocalized across the hexagonal atomic sheets of carbon contribute the graphite's conductivity. DiamondsDiamonds • Diamonds typically crystallize in the cubic crystal system and consist of tetrahedrally bonded carbon atoms • The tetrahedral arrangement of atoms in a diamond crystal is the source of many of diamond's properties; graphite, has a rhombohedral crystal structure and as a result shows dramatically different physical characteristics—contrary to diamond, graphite is a very soft, dark grey, opaque mineral. HistoryHistory ofof CarbonCarbon NanotubesNanotubes • In 1985 a new class of molecules consisting of purely carbon was discovered, the Fullerenes. -
Carbon Allotropes and Their Properties
Carbon Allotropes And Their Properties Munroe is unheaded and gangs reshuffling as necked Kristos ledger irreclaimably and politicised singly. Capitulary or unhinged, Humbert never nurtured any tootles! Crocodilian and desiccate Adams psychologised her frontage blungers trapping and raids vacillatingly. Such that transports oxygen throughout the result in drug delivery, it is decreased during the different behaviors of fullerenes, graphite and graphite The allotrope can range, was an expert in an introduction to learn how effectively utilized when a wide applications in color is dark gray selenium on. Allotrope of carbon in a property of metallic or grain boundaries within these common. Low heat resistant to their properties tailored towards their cage shape to give a property known allotropes that they be distinguished from? The property can bond lengths, their resistance to hexagonal system behaves like. The allotropes vary depending on their vat by pyrolysis and electrical properties? The allotropes background: synthesis and their properties and carbon allotropes? They are those of carbons in other. Pregnancy and carbon allotropes, from several almanacs loaded with exclusive properties to life on your use bookmark feature extraction and calcium table as. As their geological significance in? The carbon is made by their molecular structure differs from aqueous solutions program, such an error occurred. In family table salt on the sketched cells that interests to create a football shape when exposed to function in the past decade, three allotropes are. The allotropes of their properties and carbon allotropes? Carbon allotropes background: carbon when carbon allotropes that their properties. They may affect only. The allotropes of their physicochemical process and security practice question of this makes use by compressing and voidites and discovery of carbon atoms in humans burn in? You have their properties can be added in graphite, volcanoes release tons of carbons in fire door. -
Exploring Allotropes of Carbon
NNCI—Nanoscale Science and Engineering Exploring Allotropes of Carbon Explore! 1. Look at the models of diamond and graphite (both are made of carbon atoms) How do they differ? A. Does shape explain how each functions? 2. Now look at the buckyball and the carbon nanotube. How do they differ? 3. Can you roll the graphene models to form the three forms of CNTs? A. armchair, zigzag, and chiral What is an allotrope of carbon? An allotrope is a different physical form of the same material—in this case carbon. All of the models you observed are pure forms of carbons but notice how different their forms are. Carbon can have several different physical forms with the most common ones being diamond and graphite. Scientists have determined that there are other forms of pure carbon in particular buckyballs or fullerenes and carbon nanotubes (CNT). A buckyball consists of 60 carbon atoms and has a diameter of ~1.1nm while a CNT can be several nm wide and up to several mm long. These nanoscale materials have unique properties — CNTs have extraordinary mechanical, electrical, thermal, optical and chemical properties. A single CNT is 200X stronger, 5X more elastic, and 5X more conductive than steel. Visit www.nnci.net NNCI—Nanoscale Science and Engineering Exploring Allotropes of Carbon Possible applications of buckyballs include: 1. Used as a storage device for hydrogen in fuel cells. 2. Treat HIV by attaching them on to the HIV molecule section that binds to proteins thereby inhibiting the virus. 3. Their antioxidant properties may be able to fight motor deterioration caused by multiple sclerosis. -
Fullerene: Properties, Synthesis and Application
Research & Reviews: Journal of Physics ISSN: 2278-2265 (Online), ISSN: 2347-9973 (Print) Volume 6, Issue 3 www.stmjournals.com Fullerene: Properties, Synthesis and Application Jyoti Yadav* Department of Physics, Indira Gandhi University, Meerpur, Rewari, Haryana, India Abstract In this paper, a detailed study of fullerene is presented. Fullerene is the allotrope of carbon. These are also called as buckyballs. Their properties, synthesis and applications are discussed in this paper. Fullerene synthesis is done by arc discharge method and analysis is done by high performance liquid chromatography (HPLC). Synthesis process shows that C60 and C70 are produced at 1000℃ and that the concentration increases as pulse duration increases. The fullerenes can be utilized in organic photovoltaic (OPV), portable power, medical purpose, antioxidants and biopharmaceuticals. Keywords: Allotrope, hybridization, aromaticity, bonding, nanotechnology *Author for Correspondence E-mail: [email protected] INTRODUCTION corresponds to individual molecules C60 and Carbon has many allotropes like diamond, C70. The C60 molecule is a truncated graphite, etc. Third allotropic form of carbon icosahedron with an Ih symmetry while C70 has is fullerene. Any molecule made of only a more extended structure. In 1985, a new carbon atoms is fullerene. These can be of any allotrope of carbon was discovered having shape like ellipsoid, sphere or tube. Fullerenes atomic arrangement in closed shells. This having spherical geometry are called allotrope has truncated icosahedrons structure. buckyballs. Cylindrical fullerenes are given Buckminsterfullerene name was given to this the name nanotubes. Graphite has layered allotrope after the name of architect structure in which hexagonal rings are linked Buckminster Fuller who fabricated cage like to each other. -
8 Allotropes of Carbon Diamonds
8 Allotropes of Carbon DIAMONDs are forever! ¾Eachblh blac kbk ball lli is a carb on atom ¾It tends to form a cubic lattice ¾It’s the har des t known nat ural mineral ¾It has a high dispersion of light ¾80% of mined diamonds are for industrial use as they are not of jewelry quality. ¾What do they use them for in industry? Carbon doesn’t always form into the shape of a cubic lattice. • In Fact , there are 8 different allotropes of carbon. • In Greek: “to draw/write” Graphite • A conductor • The most stable • Is used in pencils • Carbon forms in Hexagonal planes Lonsdaleite • Identified in 1967 in a meteorite found in an Arizonan crater • Beeeedtobelieved to be originally graphite • due to the heat and pressure upon impact, it turned to diamond but kept the crystal structure of graphite FULLERENES • Discovered 1985 by a team of scientists from the US and England • Shape of a hollow sphere • Referred to as “buckyballs” • Also known as C60. Why? • Use nano-technology to combine the atoms in this way • Hoppgeful for use in tackling melanoma and other tough cancers C540 allotrope • Like all fullerenes, C540 is a hollow spherical shape created using nanotechnology. • How many atoms of carbon are in it? C70 • AthAnother Nanotech creation • What do all Fullerenes have in common? Amorphous Carbon (Coal & soot) • Does not have any visible crystal structure or atomic arrangement of carbon atoms • Is found containing crystallites of graphite anddid diamond d( (so small , it’s often nano size) • Coal has different grades ranging from 55% - 90% pure