Xavier-Bacalla-Thesis.Pdf
Total Page:16
File Type:pdf, Size:1020Kb
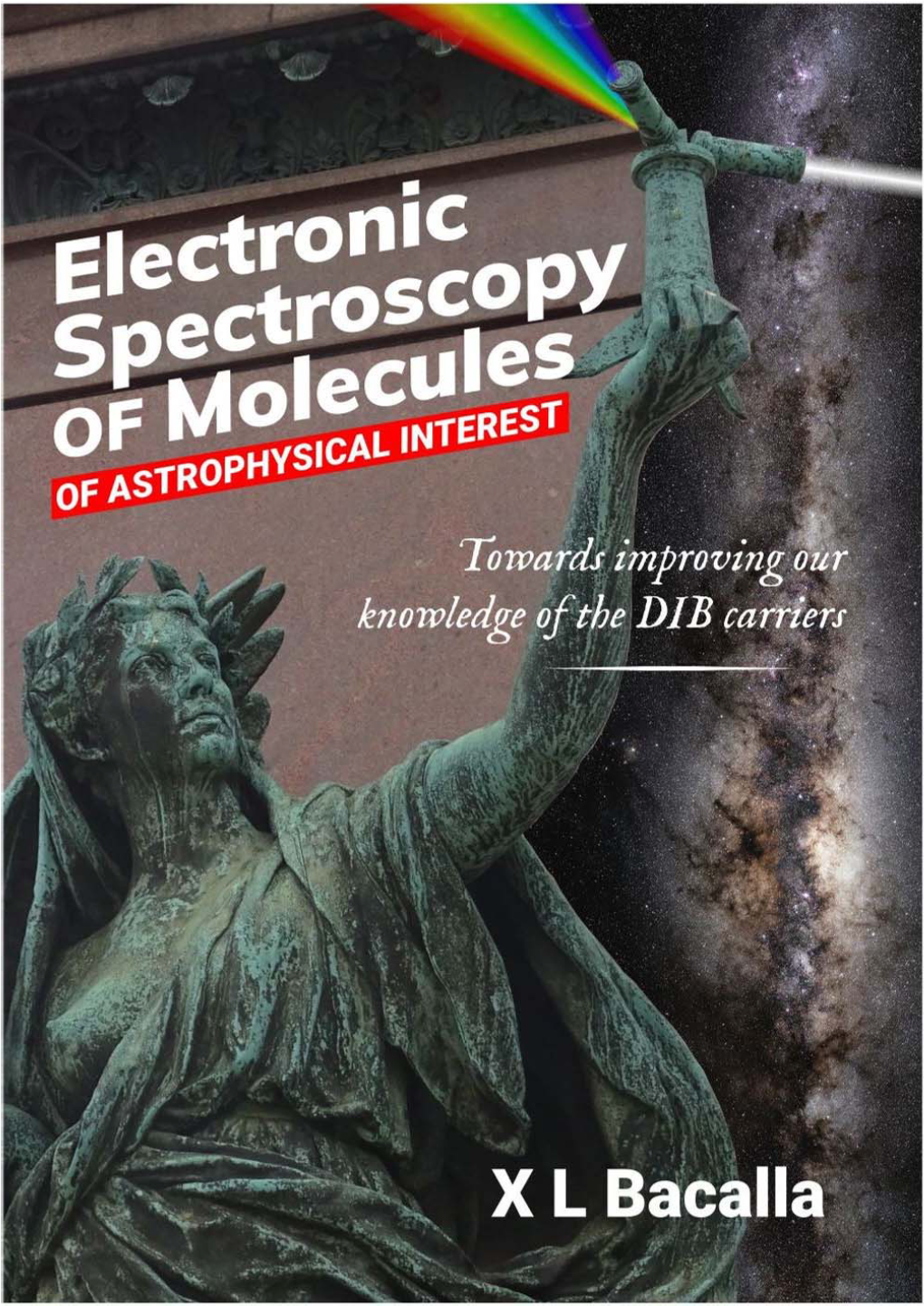
Load more
Recommended publications
-
2. Molecular Stucture/Basic Spectroscopy the Electromagnetic Spectrum
2. Molecular stucture/Basic spectroscopy The electromagnetic spectrum Spectral region fooatocadr atomic and molecular spectroscopy E. Hecht (2nd Ed.) Optics, Addison-Wesley Publishing Company,1987 Per-Erik Bengtsson Spectral regions Mo lecu lar spec troscopy o ften dea ls w ith ra dia tion in the ultraviolet (UV), visible, and infrared (IR) spectltral reg ions. • The visible region is from 400 nm – 700 nm • The ultraviolet region is below 400 nm • The infrared region is above 700 nm. 400 nm 500 nm 600 nm 700 nm Spectroscopy: That part of science which uses emission and/or absorption of radiation to deduce atomic/molecular properties Per-Erik Bengtsson Some basics about spectroscopy E = Energy difference = c /c h = Planck's constant, 6.63 10-34 Js ergy nn = Frequency E hn = h/hc /l E = h = hc / c = Velocity of light, 3.0 108 m/s = Wavelength 0 Often the wave number, , is used to express energy. The unit is cm-1. = E / hc = 1/ Example The energy difference between two states in the OH-molecule is 35714 cm-1. Which wavelength is needed to excite the molecule? Answer = 1/ =35714 cm -1 = 1/ = 280 nm. Other ways of expressing this energy: E = hc/ = 656.5 10-19 J E / h = c/ = 9.7 1014 Hz Per-Erik Bengtsson Species in combustion Combustion involves a large number of species Atoms oxygen (O), hydrogen (H), etc. formed by dissociation at high temperatures Diatomic molecules nitrogen (N2), oxygen (O2) carbon monoxide (CO), hydrogen (H2) nitr icoxide (NO), hy droxy l (OH), CH, e tc. -
Ijmp.Jor.Br V
INDEPENDENT JOURNAL OF MANAGEMENT & PRODUCTION (IJM&P) http://www.ijmp.jor.br v. 10, n. 8, Special Edition Seng 2019 ISSN: 2236-269X DOI: 10.14807/ijmp.v10i8.1046 A NEW HYPOTHESIS ABOUT THE NUCLEAR HYDROGEN STRUCTURE Relly Victoria Virgil Petrescu IFToMM, Romania E-mail: [email protected] Raffaella Aversa University of Naples, Italy E-mail: [email protected] Antonio Apicella University of Naples, Italy E-mail: [email protected] Taher M. Abu-Lebdeh North Carolina A and T State Univesity, United States E-mail: [email protected] Florian Ion Tiberiu Petrescu IFToMM, Romania E-mail: [email protected] Submission: 5/3/2019 Accept: 5/20/2019 ABSTRACT In other papers already presented on the structure and dimensions of elemental hydrogen, the elementary particle dynamics was taken into account in order to be able to determine the size of the hydrogen. This new work, one comes back with a new dynamic hypothesis designed to fundamentally change again the dynamic particle size due to the impulse influence of the particle. Until now it has been assumed that the impulse of an elementary particle is equal to the mass of the particle multiplied by its velocity, but in reality, the impulse definition is different, which is derived from the translational kinetic energy in a rapport of its velocity. This produces an additional condensation of matter in its elemental form. Keywords: Particle structure; Impulse; Condensed matter. [http://creativecommons.org/licenses/by/3.0/us/] Licensed under a Creative Commons Attribution 3.0 United States License 1749 INDEPENDENT JOURNAL OF MANAGEMENT & PRODUCTION (IJM&P) http://www.ijmp.jor.br v. -
Chemical Formulas the Elements
Chemical Formulas A chemical formula gives the numbers and types of atoms that are found in a substance. When the substance is a discrete molecule, then the chemical formula is also its molecular formula. Fe (iron) is a chemical formula Fe2O3 is a molecular formula The Elements The chemical formulas of most of the elements are simply their elemental symbol: Na (sodium) Fe (iron) He (helium) U (uranium) These chemical formulas are said to be monatomic—only an atom in chemical formula 1 The Elements There are seven elements that occur naturally as diatomic molecules—molecules that contain two atoms: H2 (hydrogen) N2 (nitrogen) O2 (oxygen) F2 (fluorine) Cl2 (chlorine) Br2 (bromine) I2 (iodine) The last four elements in this list are in the same family of the Periodic Table Binary Compounds A binary compound is one composed of only two different types of atoms. Rules for binary compound formulas 1. Element to left in Periodic Table comes first except for hydrogen: KCl PCl3 Al2S3 Fe3O4 2 Binary Compounds 2. Hydrogen comes last unless other element is from group 16 or 17: LiH, NH3, B2H4, CH4 3. If both elements are from the same group, the lower element comes first: SiC, BrF3 Other Compounds For compounds with three or more elements that are not ionic, if it contains carbon, this comes first followed by hydrogen. Other elements are then listed in alphabetical order: C2H6O C4H9BrO CH3Cl C8H10N4O2 3 Other Compounds However, the preceding rule is often ignored when writing organic formulas (molecules containing carbon, hydrogen, and maybe other elements) in order to give a better idea of how the atoms are connected: C2H6O is the molecular formula for ethanol, but nobody ever writes it this way—instead the formula is written C2H5OH to indicate one H atom is connected to the O atom. -
Syllabus of Biochemistry (Hons.)
Syllabus of Biochemistry (Hons.) for SEM-I & SEM-II under CBCS (to be effective from Academic Year: 2017-18) The University of Burdwan Burdwan, West Bengal 1 1. Introduction The syllabus for Biochemistry at undergraduate level using the Choice Based Credit system has been framed in compliance with model syllabus given by UGC. The main objective of framing this new syllabus is to give the students a holistic understanding of the subject giving substantial weight age to both the core content and techniques used in Biochemistry. The ultimate goal of the syllabus is that the students at the end are able to secure a job. Keeping in mind and in tune with the changing nature of the subject, adequate emphasis has been given on new techniques of mapping and understanding of the subject. The syllabus has also been framed in such a way that the basic skills of subject are taught to the students, and everyone might not need to go for higher studies and the scope of securing a job after graduation will increase. It is essential that Biochemistry students select their general electives courses from Chemistry, Physics, Mathematics and/or any branch of Life Sciences disciplines. While the syllabus is in compliance with UGC model curriculum, it is necessary that Biochemistry students should learn “Basic Microbiology” as one of the core courses rather than as elective while. Course on “Concept of Genetics” has been moved to electives. Also, it is recommended that two elective courses namely Nutritional Biochemistry and Advanced Biochemistry may be made compulsory. 2 Type of Courses Number of Courses Course type Description B. -
GANPAT UNIVERSITY FACULTY of SCIENCE TEACHING and EXAMINATION SCHEME Programme Bachelor of Science Branch/Spec
GANPAT UNIVERSITY FACULTY OF SCIENCE TEACHING AND EXAMINATION SCHEME Programme Bachelor of Science Branch/Spec. Microbiology Semester I Effective from Academic Year 2015- Effective for the batch Admitted in June 2015 16 Teaching scheme Examination scheme (Marks) Subject Subject Name Credit Hours (per week) Theory Practical Code Lecture(DT) Practical(Lab.) Lecture(DT) Practical(Lab.) CE SEE Total CE SEE Total L TU Total P TW Total L TU Total P TW Total UMBA101FOM FUNDAMENTALS 04 - 04 - - - 04 - 04 - - - 40 60 100 - - - OF MICROBIOLOGY UCHA101GCH GENERAL 04 - 04 - - - 04 - 04 - - - 40 60 100 - - - CHEMISTRY-I UPHA101GPH GENERAL 04 - 04 - - - 04 - 04 - - - 40 60 100 - - - PHYSICS-I UENA101ENG ENGLISH-I 02 - 02 - - - 02 - 02 - - - 40 60 100 - - - OPEN SUBJECT – 1 02 - 02 - - - 02 - 02 - - - 40 60 100 - - - UPMA101PRA PRACTICAL - - - 02 - 02 - - - 04 - 04 - - - - 50 50 MODULE-I UPCA101PRA PRACTICAL - - - 02 - 02 - - - 04 - 04 - - - - 50 50 MODULE-I UPPA101PRA PRACTICAL - - - 02 - 02 - - - 04 - 04 - - - - 50 50 MODULE-I Total 16 - 16 06 - 06 16 - 16 12 - 12 200 300 500 - 150 150 GANPAT UNIVERSITY FACULTY OF SCIENCE Programme Bachelor of Science Branch/Spec. Microbiology Semester I Version 1.0.1.0 Effective from Academic Year 2015-16 Effective for the batch Admitted in July 2015 Subject code UMBA 101 Subject Name FUNDAMENTALS OF MICROBIOLOGY FOM Teaching scheme Examination scheme (Marks) (Per week) Lecture(DT) Practical(Lab.) Total CE SEE Total L TU P TW Credit 04 -- -- -- 04 Theory 40 60 100 Hours 04 -- -- -- 04 Practical -- -- -- Pre-requisites: Students should have basic knowledge of Microorganisms and microscopy of 10+2 level. Learning Outcome: The course will help the student to understand basic fundamentals of Microbiology and history of Microbiology. -
Chemistry in One Dimension Pierre-Fran¸Coisloos,1, A) Caleb J
Chemistry in One Dimension Pierre-Fran¸coisLoos,1, a) Caleb J. Ball,1 and Peter M. W. Gill1, b) Research School of Chemistry, Australian National University, Canberra ACT 0200, Australia We report benchmark results for one-dimensional (1D) atomic and molecular sys- tems interacting via the Coulomb operator jxj−1. Using various wavefunction-type approaches, such as Hartree-Fock theory, second- and third-order Møller-Plesset per- turbation theory and explicitly correlated calculations, we study the ground state of atoms with up to ten electrons as well as small diatomic and triatomic molecules containing up to two electrons. A detailed analysis of the 1D helium-like ions is given and the expression of the high-density correlation energy is reported. We report the total energies, ionization energies, electron affinities and other interesting properties of the many-electron 1D atoms and, based on these results, we construct the 1D analog of Mendeleev's periodic table. We find that the 1D periodic table contains only two groups: the alkali metals and the noble gases. We also calculate the dissociation + 2+ 3+ curves of various 1D diatomics and study the chemical bond in H2 , HeH , He2 , + 2+ H2, HeH and He2 . We find that, unlike their 3D counterparts, 1D molecules are + primarily bound by one-electron bonds. Finally, we study the chemistry of H3 and we discuss the stability of the 1D polymer resulting from an infinite chain of hydrogen atoms. PACS numbers: 31.15.A-, 31.15.V-, 31.15.xt Keywords: one-dimensional atom; one-dimensional molecule; one-dimensional polymer; helium-like ions arXiv:1406.1343v1 [physics.chem-ph] 5 Jun 2014 a)Electronic mail: Corresponding author: [email protected] b)Electronic mail: [email protected] 1 I. -
Laser Cooling and Slowing of a Diatomic Molecule John F
Abstract Laser cooling and slowing of a diatomic molecule John F. Barry 2013 Laser cooling and trapping are central to modern atomic physics. It has been roughly three decades since laser cooling techniques produced ultracold atoms, leading to rapid advances in a vast array of fields and a number of Nobel prizes. Prior to the work presented in this thesis, laser cooling had not yet been extended to molecules because of their complex internal structure. However, this complex- ity makes molecules potentially useful for a wide range of applications. The first direct laser cooling of a molecule and further results we present here provide a new route to ultracold temperatures for molecules. In particular, these methods bridge the gap between ultracold temperatures and the approximately 1 kelvin temperatures attainable with directly cooled molecules (e.g. with cryogenic buffer gas cooling or decelerated supersonic beams). Using the carefully chosen molecule strontium monofluoride (SrF), decays to unwanted vibrational states are suppressed. Driving a transition with rotational quantum number R=1 to an excited state with R0=0 eliminates decays to unwanted ro- tational states. The dark ground-state Zeeman sublevels present in this specific scheme are remixed via a static magnetic field. Using three lasers for this scheme, a given molecule should undergo an average of approximately 100; 000 photon absorption/emission cycles before being lost via un- wanted decays. This number of cycles should be sufficient to load a magneto-optical trap (MOT) of molecules. In this thesis, we demonstrate transverse cooling of an SrF beam, in both Doppler and a Sisyphus-type cooling regimes. -
7 Spectroscopy of N2
Physics 272 Laboratory Experiments Dr. Greg Severn Spring 2016 7 Spectroscopy of N2 wax lyrical about how all chemistry arises from the fun- damentals of physics, and how biology arises from the 7.1 Introduction fundamentals of chemistry, and so on, and so forth. But this reductionist point of view is too simple, and it al- Emission spectroscopy of molecular nitrogen is the final most blinds us to the possibility of unexpected emergent spectroscopy experiment of the semester. We examine features arising in systems of increasing complexity, fea- a quantum system which comprises two atoms: the di- tures that make it difficult to untangle physics and chem- atomic molecule. We will consider the “simplest” type istry. Whats more, well find that the distinction between of molecule, the homonuclear diatomic molecule. We will simplicity and complexity becomes blurred too. We will use familiar potential energy curves and quantized en- try to see that the simple study of diatomic molecules ergy levels to understand physical characteristics of the introduces us to a system of almost bewildering complex- diatomic molecule, such as the strength of the chem- ity, and that, even in the midst of that virtual forest of ical bond, the fundamental vibrational frequency, and spectral features, we will meet the very first and simplest the mean distance between the atoms. Each of these quantum system ever solved, that of the simple harmonic characteristics depend on the electronic energy state of oscillator. the molecule. Transitions between electronic states give We will look for the simplicity within complexity. A rise to the spectra exhibited by homonuclear diatomic simple way of thinking about how molecules form is de- molecules, and we will try to connect these directly to picted in figure 2. -
Spectroscopic Study of Methane in the Broadened
SPECTROSCOPIC STUDY OF METHANE IN THE + BAND BROADENED BY ITSELF, AIR AND HYDROGEN MD ARIFUZZAMAN Master of Science, Shah Jalal University of Science and Technology, 2009 A Thesis Submitted to the School of Graduate Studies of the University of Lethbridge in Partial Fulfillment of the Requirements for the Degree MASTER OF SCIENCE Department of Physics and Astronomy University of Lethbridge LETHBRIDGE, ALBERTA, CANADA © Md Arifuzzaman, 2018 SPECTROSCOPIC STUDY OF METHANE IN THE + BAND BROADENED BY ITSELF, AIR AND HYDROGEN MD ARIFUZZAMAN Date of defence: April 27, 2018 Dr. M. Gerken Professor Ph.D. Thesis Supervisor Dr. B. Billinghurst Adjunct Assistant Professor Ph.D. Thesis Examination Committee Member Senior Scientist, Canadian Light Source. Inc. Saskatoon Dr. B. Seyed-Mahmoud Associate Professor Ph.D. Thesis Examination Committee Member Dr. A. Cross Adjunct Assistant Professor Ph.D. Thesis Examination Committee Member Dr. G. Tabisz Professor and Senior Scholar Ph.D. External Examiner Department of Physics and Astronomy University of Manitoba, Canada Dr. D. Naylor Professor Ph.D. Chair, Thesis Examination Committee Dedication This thesis is dedicated to my beloved parents, my wife and my daughter. iii Abstract This thesis presents the temperature-dependent line-shape studies of methane broadened by itself, air and hydrogen, which is identified as the second most important anthropogenic greenhouse gas in the Earth’s atmosphere due to its high global warming potential. A set of 14 laboratory spectra of pure methane and lean mixtures of methane in air were recorded over a range of temperatures from 148.4 to 298.4 K and total sample pressures from 4.5 to 385 Torr using a high-resolution Fourier Transform Spectrometer (FTS) at the Jet Propulsion Laboratory (JPL), California. -
Chemical and Dynamical Processes in the Atmospheres of I. Ancient and Present-Day Earth II
Chemical and Dynamical Processes in the Atmospheres of I. Ancient and Present-Day Earth II. Jupiter and Galilean Satellites III. Extrasolar \Hot Jupiters" Thesis by Mao-Chang Liang In Partial Ful¯llment of the Requirements for the Degree of Doctor of Philosophy California Institute of Technology Pasadena, California 2006 (Defended Nov 7, 2005) ii °c 2006 Mao-Chang Liang All Rights Reserved iii Acknowledgements I would like to thank my family for not stopping me from doing academic research; they completely supported my decision. (In Chinese culture, children's plans are mostly under their parents' control.) I would also like to acknowledge my master's thesis advisor, Professor Fred Lo, for teaching me how to be a good scientist and re- searcher. The major thing I learned from him is that, to be a good scientist, one has to remember that theory and experiments are equally important. For working on theory, one has to think of what experiments can support your theory; for experimentalists, to interpret the results requires a deep understanding of the background physics or theory. This clearly is seen from my Ph.D. advisors, Professors Yuk Yung and Ge- o® Blake, who work closely with theorists and experimentalists. Their great help in launching my research career has been invaluable. I would then want to thank my girlfriend, Bows Chiou, and colleagues Mark Allen, Mimi Gerstell, Xun Jiang, Anthony Lee, Chris Parkinson, Run-Lie Shia, and many others for their insightful comments/discussion on my research. Financial support from National Aeronautics and Space Administration (NASA) and National Science Foundation (NSF) are ac- knowledged. -
Infrared Spectroscopy of Molecules in the Gas Phase
Infrared Spectroscopy of Molecules in the Gas Phase Keqing Zhang A t hesis presented to the University of Waterloo in fdflment of the thesis requirement for the degree of Doctor of Philosophy in Chemistry Waterloo, Ontario, Canada. 1997 @Keqing Zhang 1997 Nationai Library Bibliothèque nationaie du Canada Acqui..itions and Acquisitions et Bibliographie Senrices services bibliographiques The author has granted a non- L'auteur a accordé une licence non exclusive licence allowing the exclusive permettant à la National LiIbrary of Canada to Bibliothèque nationale du Canada de reproduce, loan, distn'biite or sell reproduire, prêter, distriiuer ou copies of Mer thesis by any means vendre des copies de sa thèse de and in any form or format, making quelque dèreet sous queIque this thesis available to interested forme que ce soit pour mettre des pemns. exemplaires de cette thèse à la disposition des personnes intéressées. The author retains omership of the L'auteur conserve la propriété du copyright in Merthesis. Neither droit d'auieur qui protège sa thése. Ni the thesis nor substantiai extracts ia thèse ni des extraits substantiels de fkom it may be printed or otherwise celle-ci ne doivent être miprimés ou reproduced with the author's autrement reproduits sans son permission. autorisation. The University of Waterloo requires the signatures of all persons using or ph* tocopying this thesis. Please sign below, and give address and date. Abstract Fourier transforxn idiared spectroscopy is appiied to the studies of several very different moledar systems. The spectra of the diatomic moledes BF, ALF, and MgF were recorded and analyzed. -
9.5 Rotation and Vibration of Diatomic Molecules
9.5. Rotation and Vibration of Diatomic Molecules 349 9.5 Rotation and Vibration of Diatomic Molecules Up to now we have discussed the electronic states of ri- gid molecules, where the nuclei are clamped to a fixed position. In this section we will improve our model of molecules and include the rotation and vibration of dia- tomic molecules. This means, that we have to take into account the kinetic energy of the nuclei in the Schrö- dinger equation Hψ Eψ, which has been omitted in ˆ the foregoing sections=. We then obtain the Hamiltonian 2 2 1 2 N H ! 2 ! 2 ˆ = − 2 M ∇k − 2m ∇i k 1 k e i 1 != != 2 e Z1 Z2 1 1 1 el Fig. 9.41. Energy En (R) of the rigid molecule and total + 4πε R + r − r + r energy E of the nonrigid vibrating and rotating molecule 0 i, j i, j i i1 i2 ! ! $ % Enucl Eel E0 T H (9.73) ˆkin kin pot ˆk ˆ0 = + + = + can be written as the product of the molecular wave where the first term represents the kinetic energy of the function χ(Rk) (which depends on the positions Rk of nuclei, the second term that of the electrons, the third the nuclei), and the electronic wave function Φ(ri , Rk) represents the potential energy of nuclear repulsion, the of the rigid molecule at arbitrary but fixed nuclear po- fourth that of the electron repulsion and the last term sitions Rk, where the electron coordinates ri are the the attraction between the electrons and the nuclei.