Plasmolysis: Loss of Turgor and Beyond
Total Page:16
File Type:pdf, Size:1020Kb
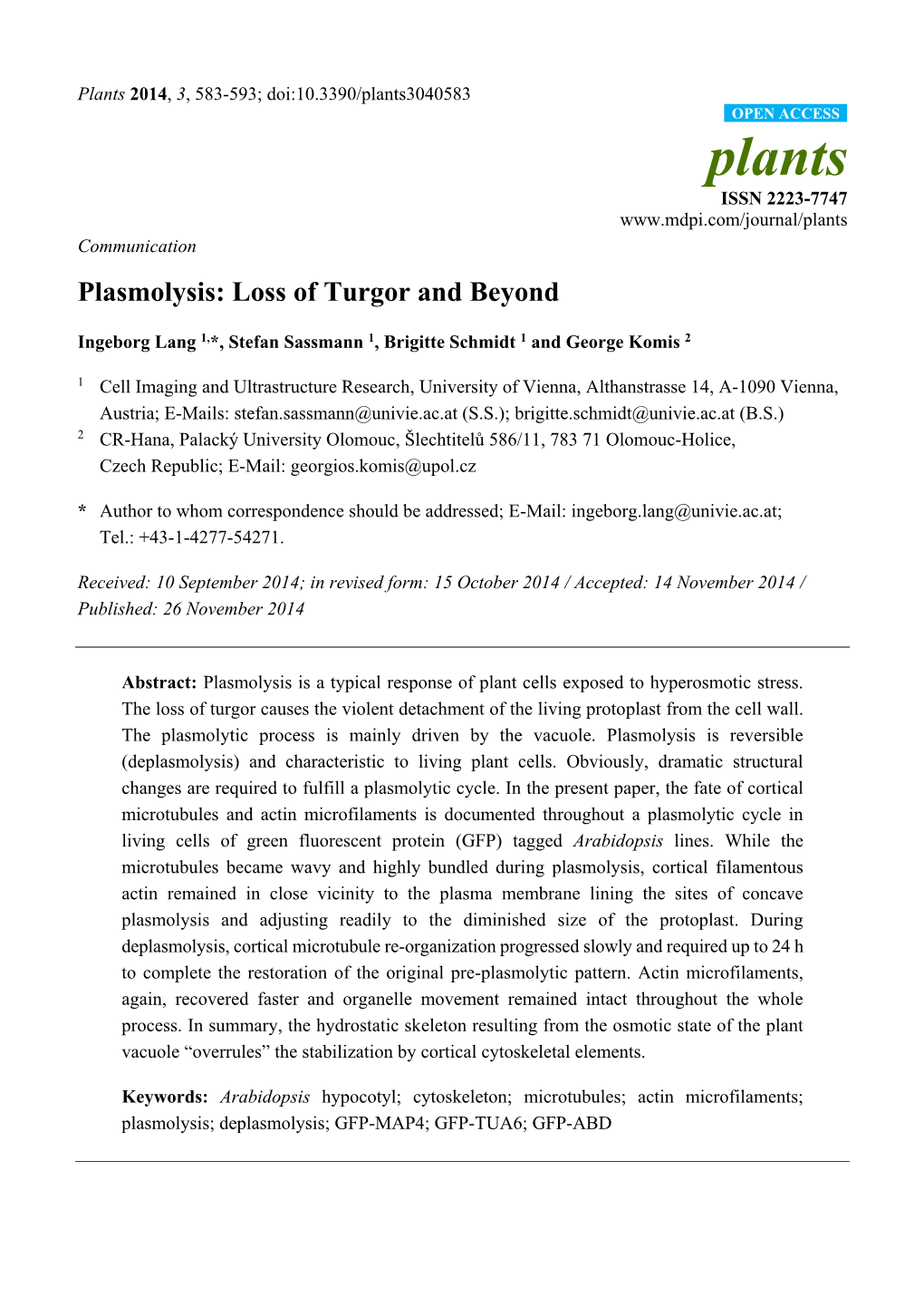
Load more
Recommended publications
-
Revised Glossary for AQA GCSE Biology Student Book
Biology Glossary amino acids small molecules from which proteins are A built abiotic factor physical or non-living conditions amylase a digestive enzyme (carbohydrase) that that affect the distribution of a population in an breaks down starch ecosystem, such as light, temperature, soil pH anaerobic respiration respiration without using absorption the process by which soluble products oxygen of digestion move into the blood from the small intestine antibacterial chemicals chemicals produced by plants as a defence mechanism; the amount abstinence method of contraception whereby the produced will increase if the plant is under attack couple refrains from intercourse, particularly when an egg might be in the oviduct antibiotic e.g. penicillin; medicines that work inside the body to kill bacterial pathogens accommodation ability of the eyes to change focus antibody protein normally present in the body acid rain rain water which is made more acidic by or produced in response to an antigen, which it pollutant gases neutralises, thus producing an immune response active site the place on an enzyme where the antimicrobial resistance (AMR) an increasing substrate molecule binds problem in the twenty-first century whereby active transport in active transport, cells use energy bacteria have evolved to develop resistance against to transport substances through cell membranes antibiotics due to their overuse against a concentration gradient antiretroviral drugs drugs used to treat HIV adaptation features that organisms have to help infections; they -
Penetration of Hard Substrates by a Fungus Employing Enormous Turgor Pressures (Appressorium/Biodeterioration/Magnaporthe Gnsea/Plant Pathogen/Rice Blast) RICHARD J
Proc. Natd. Acad. Sci. USA Vol. 88, pp. 11281-11284, December 1991 Microbiology Penetration of hard substrates by a fungus employing enormous turgor pressures (appressorium/biodeterioration/Magnaporthe gnsea/plant pathogen/rice blast) RICHARD J. HOWARD*t, MARGARET A. FERRARI*, DAVID H. ROACHt, AND NICHOLAS P. MONEY§ *Central Research and Development, and tFibers, The DuPont Company, Wilmington, DE 19880-0402; and §Department of Biochemistry, Colorado State University, Fort Collins, CO 80523 Communicated by Arthur Kelman, September 20, 1991 (receivedfor review June 27, 1991) ABSTRACT Many fungal pathogens penetrate plant MATERIALS AND METHODS an The rice leaves from a specialized cell called appressorium. Organism and Growth Conditions. These studies were blast pathogen Magnaporthegnsea can also penetrate synthetic conducted with strain 042 (see ref. 8) of M. grisea (Hebert) surfaces such as poly(vinyl chloride). Previous experiments time requires an elevated appres- Barr, telomorph of Pyricularia grisea Sacc. (10). The have suggested that penetration course of infection-structure development in vitro has been sorial turgor pressure. In the present report we have used well documented and closely resembles development on the nonbiodegradable Mylar membranes, exhibiting a range of in that penetration is host (11, 12). When harvested and placed on a surface surface hardness, to test the proposition distilled water, conidia germinate in 1-3 hr to form germ driven by turgor. Reducing appressorial turgor by osmotic to form and are firmly stress inhibited penetration ofthese membranes. The size ofthe tubes. By 4 hr appressoria begin was a function of attached to the substratum. By 6-8 hr their structure appears turgor deficit required to inhibit penetration complete. -
What Is Elodea? Elodea Cell Elodea Cell Parts
What is Elodea? • The Elodea plant has Plasmolysis the parts that would typically be and found in a plant cell. Cytolysis In this exercise we will identify those parts and see what happens when you subject this plant to plasmolysis. Elodea Cell Elodea Cell Parts It is not possible to see the cell membrane because it is pushed up against the cell wall. Also, the central vacuole • Name three structures that are cannot be clearly distinguished from the cell cytoplasm. shown in this plant cell that In a living elodea cell you can “roughly” tell where the you would not expect to find in vacuole is by where the moving chloroplasts are not able an animal cell? to go. 1 What is plasmolysis? Elodea Plasmolysis • If you were to surround this group of plant cells with salt water then the water inside the plant would move from where there is • This is a view of a cell more water (less salt) through the cell wall and membrane to the that has undergone outside where there is less water (more salt). This process of water movement from a high concentration of water to a lesser plasmolysis. It is now concentration of water is called osmosis. When the water possible to see the movement is out from a cell this form of osmosis is specifically called plasmolysis. cytoplasm which has contracted around the chloroplasts and the other cellular structures. Most of the water that has left the cell has been from the vacuole. Onion Skin Plasmolysis Types of Solutions Hypertonic solutions contain higher concentrations of solutes than those in surrounding cells resulting in the cell shrinking in size. -
An Analysis of the Water Potential Isotherm in Plant Tissue
AN ANALYSIS OF THE WATER POTENTIAL ISOTHERM IN PLANT TISSUE 11.* COMPARATIVE STUDIES ON LEAVES OF DIFFERENT TYPES By 1. Noy-MEIRH and B. Z. GINZBURGt [Manu8cript received January 26, 1968] Summary The water potential isotherms of leaves of carob (a sclerophyllic xerophyte), plane tree (a mesophyte), and saltbush (a semisucculent xero-halophyte) were measured by vapour equilibration with filter paper. The isotherm of the living tissue was partitioned into components by measuring the isotherms of killed tissue and of isolated matrix fractions. Empirical functions were fitted by regression to each of the components. The isotherm of the matrix fractions fitted best to a function of the form 'P = -a/w2 +b/w and the isotherms of killed tissue, whether before or after subtraction of the matrix, to a function of the form 'P = -a/w2 -b/w. The first term indicates non-ideality of the tissue solution. The water potential difference between living and killed tissue, which is an approximation of the hydro static potential, was far from linear with water content; either a quadratic function or two discontinuous linear ones could be fitted to it. Negative hydrostatic poten tials were measured, the highest values (20 atm) being attained in carob. A hyster etic component was measured both in the entire tissue and in the matrix fractions. The parameters of the isotherm (the coefficients of the functions) for the leaves of the three species were compared and related to their "drought tolerance" and their ecological preferences. The leaves of the two xerophytes, carob and salt bush, can both tolerate lower water potentials than those of plane, but have very different isotherms. -
Cell Membrane & Tonicity Worksheet
NAME__________ ANSWER KEY ___________________ DATE_________________ PERIOD_________ Cell Membrane & Tonicity Worksheet Composition of the Cell Membrane & Functions The cell membrane is also called the PLASMA membrane and is made of a phospholipid SKETCH AND LABEL a phospholipid coloring BI-LAYER. The phospholipids have a hydrophilic (water attracting) HEADS and two the heads red and the hydrophobic (water repelling) TAILS. The head of a phospholipid is made of an alcohol and tails blue. GLYCEROL group, while the tails are chains of FATTY ACIDS. Phospholipids can move SIDEWAYS and allow water and other NON-POLAR molecules to pass through into or out of the cell. This is known as simple PASSIVE TRANSPORT because it does not require ENERGY and the water or molecules are moving WITH the concentration gradient. Another type of lipid in the cell membrane is CHOLESTEROL that makes the membrane more fluid. Embedded in the phospholipid bilayer are PROTEINS that also aid in diffusion and in cell recognition. Proteins called INTEGRAL proteins go all the way through the bilayer, while PERIPHERAL proteins are only on one side. Large molecules like PROTEINS or carbohydrates use proteins to help move across cell membranes. Some of the membrane proteins have carbohydrate PARTS attached to help cells in recognize each other and certain molecules. List 4 functions of the cell or plasma membrane: a. CELL SIGNALING b. SELECTIVE TRANSPORT c. EXCRETION OF WASTES d. STRUCTURAL SUPPORT Correctly color code and identify the name for each part of the cell membrane. Letter Name/Color Letter Name/Color __ A ___ Phospholipid bilayer (no color) __ H ___ Peripheral protein (red) __ B ___ Integral protein (pink) __ I ____ Cholesterol (blue) __ F ___ Fatty acid tails (orange) __ C___ Glycoprotein (green) __ G ___ Phosphate heads (yellow) __ E ___ Glycolipids (purple) Match the cell membrane structure or its function with the correct letter from the cell membrane diagram. -
Volume 1, Chapter 7-5: Water Relations: Physiological Adaptations
Glime, J. M. 2017. Water Relations: Physiological Adaptations. Chapt. 7-5. In: Glime, J. M. Bryophyte Ecology. Volume 1. 7-5-1 Physiological Ecology. Ebook sponsored by Michigan Technological University and the International Association of Bryologists. Last updated 17 July 2020 and available at <http://digitalcommons.mtu.edu/bryophyte-ecology/>. CHAPTER 7-5 WATER RELATIONS: PHYSIOLOGICAL ADAPTATIONS TABLE OF CONTENTS Water Relations on Land ..................................................................................................................................... 7-5-2 Drought Tolerance vs Avoidance ........................................................................................................................ 7-5-3 Desiccation Tolerance .................................................................................................................................. 7-5-4 Desiccation Avoidance ................................................................................................................................ 7-5-9 Life Cycle and Life Strategy Adaptations ........................................................................................................... 7-5-9 Seasonal Changes .............................................................................................................................................. 7-5-11 Physiological Adaptations ................................................................................................................................. 7-5-14 Mode of Conduction -
Hornwort Stomata Do Not Respond Actively to Exogenous and Environmental Cues
Annals of Botany 122: 45–57, 2018 doi: 10.1093/aob/mcy045, available online at www.academic.oup.com/aob Hornwort stomata do not respond actively to exogenous and environmental cues Silvia Pressel1,*, Karen S. Renzaglia2, Richard S. (Dicky) Clymo3 and Jeffrey G. Duckett1 1Life Sciences Department, Natural History Museum, Cromwell Road, London SW7 5BD, UK, 2Plant Biology Department, Southern Illinois University, Carbondale, IL 62901, USA and 3School of Biological and Chemical Sciences, Queen Mary University of London, Mile End Road, London E1 4NS, UK *For correspondence. E-mail [email protected] Downloaded from https://academic.oup.com/aob/article-abstract/122/1/45/4979633 by guest on 11 March 2019 Received: 25 October 2017 Returned for revision: 13 November 2017 Editorial decision: 16 February 2018 Accepted: 14 March 2018 Published electronically 20 April 2018 • Backgrounds and Aims Because stomata in bryophytes occur on sporangia, they are subject to different developmental and evolutionary constraints from those on leaves of tracheophytes. No conclusive experimental evidence exists on the responses of hornwort stomata to exogenous stimulation. • Methods Responses of hornwort stomata to abscisic acid (ABA), desiccation, darkness and plasmolysis were compared with those in tracheophyte leaves. Potassium ion concentrations in the guard cells and adjacent cells were analysed by X-ray microanalysis, and the ontogeny of the sporophytic intercellular spaces was compared with those of tracheophytes by cryo-scanning electron microscopy. • Key Results The apertures in hornwort stomata open early in development and thereafter remain open. In hornworts, the experimental treatments, based on measurements of >9000 stomata, produced only a slight reduction in aperture dimensions after desiccation and plasmolysis, and no changes following ABA treatments and darkness. -
Glossary.Pdf
Glossary Pronunciation Key accessory fruit A fruit, or assemblage of fruits, adaptation Inherited characteristic of an organ- Pronounce in which the fleshy parts are derived largely or ism that enhances its survival and reproduc- a- as in ace entirely from tissues other than the ovary. tion in a specific environment. – Glossary Ј Ј a/ah ash acclimatization (uh-klı¯ -muh-tı¯-za -shun) adaptive immunity A vertebrate-specific Physiological adjustment to a change in an defense that is mediated by B lymphocytes ch chose environmental factor. (B cells) and T lymphocytes (T cells). It e¯ meet acetyl CoA Acetyl coenzyme A; the entry com- exhibits specificity, memory, and self-nonself e/eh bet pound for the citric acid cycle in cellular respi- recognition. Also called acquired immunity. g game ration, formed from a fragment of pyruvate adaptive radiation Period of evolutionary change ı¯ ice attached to a coenzyme. in which groups of organisms form many new i hit acetylcholine (asЈ-uh-til-ko–Ј-le¯n) One of the species whose adaptations allow them to fill dif- ks box most common neurotransmitters; functions by ferent ecological roles in their communities. kw quick binding to receptors and altering the perme- addition rule A rule of probability stating that ng song ability of the postsynaptic membrane to specific the probability of any one of two or more mu- o- robe ions, either depolarizing or hyperpolarizing the tually exclusive events occurring can be deter- membrane. mined by adding their individual probabilities. o ox acid A substance that increases the hydrogen ion adenosine triphosphate See ATP (adenosine oy boy concentration of a solution. -
Regulation of Microbial Growth by Turgor Pressure
Available online at www.sciencedirect.com ScienceDirect Regulation of microbial growth by turgor pressure 1,2 1,3 Enrique R Rojas and Kerwyn Casey Huang Rapid changes in environmental osmolarity are a natural ultimate process that determines the rate of cell growth. aspect of microbial lifestyles. The change in turgor pressure The envelope is inflated by turgor pressure, the intracel- resulting from an osmotic shock alters the mechanical forces lular hydrostatic pressure that results from the osmotic within the cell envelope, and can impact cell growth across potential (concentration differential) across the mem- a range of timescales, through a variety of mechanical brane, which is balanced by mechanical stress in the cell mechanisms. Here, we first summarize measurements of turgor envelope (Figure 1a). Since water is the primary cytosolic pressure in various organisms. We then review how the component, and bacterial cells do not have active water combination of microfluidic flow cells and quantitative image transporters, cells rely on osmosis for water import during analysis has driven discovery of the diverse ways in which cell growth. Indeed, the idea that swelling due to osmosis turgor pressure mechanically regulates bacterial growth, is fundamental to cell growth is centuries old [2]. How- independent of the effect of cytoplasmic crowding. In Gram- ever, recent progress has aimed to understand deeper positive, rod-shaped bacteria, reductions in turgor pressure functional relationships between water activity and cell cause decreased growth rate. Moreover, a hypoosmotic shock, growth. These studies demonstrated that, in many cases, which increases turgor pressure and membrane tension, leads osmotic potential is not simply required for water influx, to transient inhibition of cell-wall growth via electrical but is required to generate turgor pressure that is used as a depolarization. -
Glossary of Biotechnology and Genetic Engineering 1
FAO Glossary of RESEARCH AND biotechnology TECHNOLOGY and PAPER genetic engineering 7 A. Zaid H.G. Hughes E. Porceddu F. Nicholas Food and Agriculture Organization of the United Nations Rome, 1999 – ii – The designations employed and the presentation of the material in this document do not imply the expression of any opinion whatsoever on the part of the United Nations or the Food and Agriculture Organization of the United Nations concerning the legal status of any country, territory, city or area or of its authorities, or concerning the delimitation of its frontiers or boundaries. ISBN: 92-5-104369-8 ISSN: 1020-0541 All rights reserved. No part of this publication may be reproduced, stored in a retrieval system, or transmitted in any form or by any means, electronic, mechanical, photocopying or otherwise, without the prior permission of the copyright owner. Applications for such permission, with a statement of the purpose and extent of the reproduction, should be addressed to the Director, Information Division, Food and Agriculture Organization of the United Nations, Viale delle Terme di Caracalla, 00100 Rome, Italy. © FAO 1999 – iii – PREFACE Biotechnology is a general term used about a very broad field of study. According to the Convention on Biological Diversity, biotechnology means: “any technological application that uses biological systems, living organisms, or derivatives thereof, to make or modify products or processes for specific use.” Interpreted in this broad sense, the definition covers many of the tools and techniques that are commonplace today in agriculture and food production. If interpreted in a narrow sense to consider only the “new” DNA, molecular biology and reproductive technology, the definition covers a range of different technologies, including gene manipulation, gene transfer, DNA typing and cloning of mammals. -
Membrane Permeability-Cell
Membrane Permeability Background Information: A defining feature of all cells is the plasma membrane that separates the interior of the cell from its external environment. The plasma membrane is composed of a lipid bilayer and a variety of proteins that are embedded in that bilayer. The lipid portion of the membrane serves a barrier function, preventing most molecules and ions from passing in or out. In order for most molecules or ions to enter or exit the cell they must pass through a channel or carrier protein in the membrane. In this way, the plasma membrane is said to be selectively permeable. The plasma membrane of most cells is freely permeable to water. The diffusion of water across a semi-permeable membrane in response to a concentration gradient is known as osmosis. Cells normally occupy an environment with a particular concentration of solutes. Usually, the internal solute concentration is equal to the external solute concentration so there is no net movement of water molecules across the membrane. Such a solution is said to be isotonic. If the solute concentration outside the cell is greater than inside the cell, the solution is said to be hypertonic; if the solute concentration outside the cell is less than that inside the cell, the solution is hypotonic. Cells in isotonic solutions maintain a relatively constant internal volume because there is no net movement of water into or out of the cell. When a cell is placed in a hypotonic solution, water will enter the cell in response to the osmotic gradient. This influx of water will cause the cell to swell and, if the concentration gradient is steep, the cell may burst, a process known as lysis. -
Biology Glossary
High School Level Biology Glossary English | Spanish Translation of Biology terms based on the Coursework for Living Environment Grades 9 to 12. Glossary This glossary is to PROVIDE PERMITTED TESTING ACCOMMODATIONS of ELL students. It also needs to be used for INSTRUCTION during the school year. The glossary may be downloaded, printed and disseminated to educators, parents and ELLs. Acknowledgements: Thank you to the New York State Education Department for sharing this powerful resource on behalf of all ELLs. THE STATE EDUCATION DEPARTMENT / THE UNIVERSITY OF THE STATE OF NEW YORK / ALBANY, NY 12234 Updated: December 2019 Living Environment Glossary English –Spanish Page 1 of 30 A adenosine diphosphate (ADP) : difosfato de adenosina (DFA) adenosine monophosphate (AMP) : abandon : abandonar monofosfato de adenosina (MFA) abbreviation : abreviatura adenosine triphosphate (ATP) : trifosfato de adenosina (TFA) ability : aptitud adequate : adecuado abiogenesis : abiogénesis adhesion : adhesión abiotic : abiótico adipose tissue : tejido adiposo abiotico factor : factor abiótico adolescence : adolescencia abortion : aborto adrenal cortex : corteza suprarrenal absorption : absorción adrenal gland : glándula suprarrenal accomplish : lograr adrenal medulla : médula suprarrenal according to : según adrenaline : adrenalina adrenocorticotropic hormone(ACTH) : account : cuenta hormona adrenocorticotrópica (HACT) accumulate : acumular adsorption : adsorción accurate : preciso adult : adulto acetylcholine : acetilcolina advantage : ventaja acid : ácido