(+)-Lactic Acid-Producing Bacterium Enterococcus Mundtii
Total Page:16
File Type:pdf, Size:1020Kb
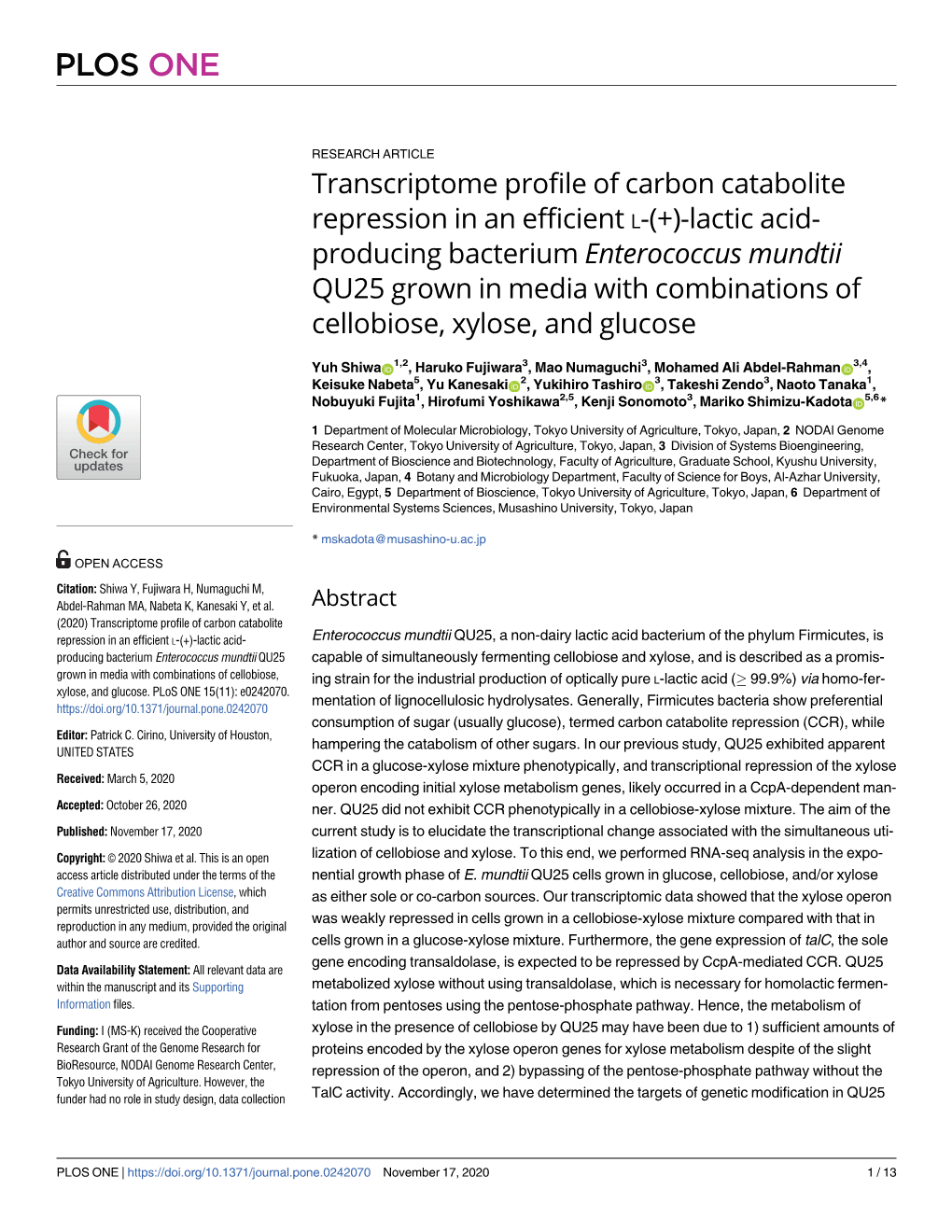
Load more
Recommended publications
-
Adaptive Strategies of Enterococcus Mundtii to Different Living Conditions in the Gut Microbiome of Spodoptera Littoralis Larvae
Adaptive strategies of Enterococcus mundtii to different living conditions in the gut microbiome of Spodoptera littoralis larvae Dissertation To Fulfill the Requirements for the Degree of „doctor rerum naturalium“ (Dr. rer. nat.) Submitted to the Council of the Faculty of Biological Sciences of the Friedrich Schiller University Jena By Tilottama Mazumdar, Masters in Biotechnology, born on 17.04.1992 in India Reviewers 1. Prof. Dr. Wilhelm Boland, Department of Bioorganic Chemistry, Max Planck Institute for Chemical Ecology 2. Prof. Dr. Erika Kothe, Institute of Microbiology, Friedrich Schiller University 3. Prof. Dr. David Heckel, Department of Entomology, Max Planck Institute for Chemical Ecology 4. Dr. Mark S Gresnigt, Leibniz Institute for Natural Product Research and Infection Biology – Hans Knöll Institute (HKI) 5. Prof. Dr. Dirk Hoffmeister, Leibniz Institute for Natural Product Research and Infection Biology – Hans Knöll Institute (HKI) 6. Prof. Dr. Dino McMahon, Institute of Biology – Zoology, Freie Universität Berlin Date of Defense- 19th November, 2020 2 “We are all of us walking communities of bacteria. The world shimmers, a pointillist landscape made of tiny living beings” --- Lynn Marguilis, Microcosmos: Four Billion Years of Microbial Evolution, 1986 “We can allow satellites, planets, suns, universe, nay whole systems of universe, to be governed by laws, but the smallest insect, we wish to be created at once by special act.” --- Charles Darwin, Darwin’s religious odessey, 2002 “Science cannot solve the ultimate mystery of nature. And that is because, in the last analysis, we ourselves are a part of the mystery that we are trying to solve.” --- Max Planck, Where is Science going? , 1981 3 Contents 1 Introduction ................................................................................................................................... -
Development and Role of the Indigenous Gut Microbiota Of
Development and role of the indigenous gut microbiota of Spodoptera littoralis Dissertation To Fulfill the Requirements for the Degree of ,,doctor rerum naturalium“ (Dr. rer. nat.) Submitted to the Council of the Faculty of Biology and Pharmacy of the Friedrich Schiller University Jena by Beng-Soon Teh (M.Sc) born on 12.02.1985 in Penang, Malaysia Gutachter: 1. …. 2. …. 3. …. Tag der öffentlichen Verteidigung: Fluorescent GFP-tagged Enterococcus mundtii TABLE of CONTENTS Abbreviations and symbols 1. Introduction .......................................................................................................... 1 1.1 Host-microbiota symbiosis interactions ........................................................... 1 1.1.1 Insect-bacteria symbiosis interactions ........................................................ 2 1.2 Physiological conditions and stresses in the gut environment of insects ......... 3 1.3 Contributions of the gut microbiome ................................................................ 5 1.4 Diversity of the gut microbiota in insects ......................................................... 6 1.5 Model organism: Spodoptera littoralis ............................................................. 9 1.6 The physiology of lactic acid bacteria ............................................................ 10 1.6.1 General characteristics of enterococci ...................................................... 11 1.7 Colonization of enterococci in insects ............................................................ 14 -
Prevalent Human Gut Bacteria Hydrolyse and Metabolise Important Food-Derived Mycotoxins and Masked Mycotoxins
toxins Article Prevalent Human Gut Bacteria Hydrolyse and Metabolise Important Food-Derived Mycotoxins and Masked Mycotoxins Noshin Daud 1, Valerie Currie 1 , Gary Duncan 1, Freda Farquharson 1, Tomoya Yoshinari 2, Petra Louis 1 and Silvia W. Gratz 1,* 1 Rowett Institute, University of Aberdeen, Foresterhill, Aberdeen AB25 2ZD, UK; [email protected] (N.D.); [email protected] (V.C.); [email protected] (G.D.); [email protected] (F.F.); [email protected] (P.L.) 2 Division of Microbiology, National Institute of Health Sciences, 3-25-26 Tonomachi, Kawasaki-ku, Kawasaki-shi, Kanagawa 210-9501, Japan; [email protected] * Correspondence: [email protected] Received: 21 September 2020; Accepted: 9 October 2020; Published: 13 October 2020 Abstract: Mycotoxins are important food contaminants that commonly co-occur with modified mycotoxins such as mycotoxin-glucosides in contaminated cereal grains. These masked mycotoxins are less toxic, but their breakdown and release of unconjugated mycotoxins has been shown by mixed gut microbiota of humans and animals. The role of different bacteria in hydrolysing mycotoxin-glucosides is unknown, and this study therefore investigated fourteen strains of human gut bacteria for their ability to break down masked mycotoxins. Individual bacterial strains were incubated anaerobically with masked mycotoxins (deoxynivalenol-3-β-glucoside, DON-Glc; nivalenol-3-β-glucoside, NIV-Glc; HT-2-β-glucoside, HT-2-Glc; diacetoxyscirpenol-α-glucoside, DAS-Glc), or unconjugated mycotoxins (DON, NIV, HT-2, T-2, and DAS) for up to 48 h. Bacterial growth, hydrolysis of mycotoxin-glucosides and further metabolism of mycotoxins were assessed. -
The Ecology, Epidemiology and Virulence of Enterococcus
Microbiology (2009), 155, 1749–1757 DOI 10.1099/mic.0.026385-0 Review The ecology, epidemiology and virulence of Enterococcus Katie Fisher and Carol Phillips Correspondence University of Northampton, School of Health, Park Campus, Boughton Green Road, Northampton Katie Fisher NN2 7AL, UK [email protected] Enterococci are Gram-positive, catalase-negative, non-spore-forming, facultative anaerobic bacteria, which usually inhabit the alimentary tract of humans in addition to being isolated from environmental and animal sources. They are able to survive a range of stresses and hostile environments, including those of extreme temperature (5–65 6C), pH (4.5”10.0) and high NaCl concentration, enabling them to colonize a wide range of niches. Virulence factors of enterococci include the extracellular protein Esp and aggregation substances (Agg), both of which aid in colonization of the host. The nosocomial pathogenicity of enterococci has emerged in recent years, as well as increasing resistance to glycopeptide antibiotics. Understanding the ecology, epidemiology and virulence of Enterococcus speciesisimportant for limiting urinary tract infections, hepatobiliary sepsis, endocarditis, surgical wound infection, bacteraemia and neonatal sepsis, and also stemming the further development of antibiotic resistance. Introduction Taxonomy For many years Enterococcus species were believed to be The genus Enterococcus consists of Gram-positive, catalase- harmless to humans and considered unimportant med- negative, non-spore-forming, facultative anaerobic bacteria ically. Because they produce bacteriocins, Enterococcus that can occur both as single cocci and in chains. species have been used widely over the last decade in the Enterococci belong to a group of organisms known as food industry as probiotics or as starter cultures (Foulquie lactic acid bacteria (LAB) that produce bacteriocins Moreno et al., 2006). -
Characterization of the Bacterial Gut Microbiota of Piglets Suffering From
Hermann-Bank et al. BMC Veterinary Research (2015) 11:139 DOI 10.1186/s12917-015-0419-4 RESEARCH ARTICLE Open Access Characterization of the bacterial gut microbiota of piglets suffering from new neonatal porcine diarrhoea Marie Louise Hermann-Bank1, Kerstin Skovgaard1, Anders Stockmarr2, Mikael Lenz Strube1, Niels Larsen3, Hanne Kongsted4, Hans-Christian Ingerslev1, Lars Mølbak1,5 and Mette Boye1* Abstract Background: In recent years, new neonatal porcine diarrhoea (NNPD) of unknown aetiology has emerged in Denmark. NNPD affects piglets during the first week of life and results in impaired welfare, decreased weight gain, and in the worst-case scenario death. Commonly used preventative interventions such as vaccination or treatment with antibiotics, have a limited effect on NNPD. Previous studies have investigated the clinical manifestations, histopathology, and to some extent, microbiological findings; however, these studies were either inconclusive or suggested that Enterococci, possibly in interaction with Escherichia coli, contribute to the aetiology of NNPD. This study examined ileal and colonic luminal contents of 50 control piglets and 52 NNPD piglets by means of the qPCR-based Gut Microbiotassay and 16 samples by 454 sequencing to study the composition of the bacterial gut microbiota in relation to NNPD. Results: NNPD was associated with a diminished quantity of bacteria from the phyla Actinobacteria and Firmicutes while genus Enterococcus was more than 24 times more abundant in diarrhoeic piglets. The number of bacteria from the phylum Fusobacteria was also doubled in piglets suffering from diarrhoea. With increasing age, the gut microbiota of NNPD affected piglet and control piglets became more diverse. Independent of diarrhoeic status, piglets from first parity sows (gilts) possessed significantly more bacteria from family Enterobacteriaceae and species E. -
Cycle 36 Organism 1
P.O. Box 131375, Bryanston, 2074 Ground Floor, Block 5 Bryanston Gate, 170 Curzon Road Bryanston, Johannesburg, South Africa 804 Flatrock, Buiten Street, Cape Town, 8001 www.thistle.co.za Tel: +27 (011) 463 3260 Fax: +27 (011) 463 3036 Fax to Email: + 27 (0) 86-557-2232 e-mail : [email protected] Please read this section first The HPCSA and the Med Tech Society have confirmed that this clinical case study, plus your routine review of your EQA reports from Thistle QA, should be documented as a “Journal Club” activity. This means that you must record those attending for CEU purposes. Thistle will not issue a certificate to cover these activities, nor send out “correct” answers to the CEU questions at the end of this case study. The Thistle QA CEU No is: MT-2014/004. Each attendee should claim THREE CEU points for completing this Quality Control Journal Club exercise, and retain a copy of the relevant Thistle QA Participation Certificate as proof of registration on a Thistle QA EQA. MICROBIOLOGY LEGEND CYCLE 36 ORGANISM 1 Enterococcus casseliflavus Enterococcus is a genus of lactic acid bacteria of the phylum Firmicutes. Enterococci are Gram-positive cocci that often occur in pairs (diplococci) or short chains, and are difficult to distinguish from streptococci on physical characteristics alone. Two species are common commensal organisms in the intestines of humans: E. faecalis (90-95%) and E. faecium (5-10%) but are also important pathogens responsible for serious infections. Rare clusters of infections occur with other species, including E. casseliflavus, E. gallinarum, and E. -
Resistance Mechanisms and Inflammatory Bowel Disease 10.1515/Med-2020-0032 Present Multi-Resistant E
Open Med. 2020; 15: 211-224 Research Article Michaela Růžičková, Monika Vítězová, Ivan Kushkevych* The characterization of Enterococcus genus: resistance mechanisms and inflammatory bowel disease https://doi.org/ 10.1515/med-2020-0032 present multi-resistant E. faecium belongs to a different received September 18, 2019; accepted February 5, 2020 taxon than the original strains isolated from animals. This separation must have happened around 75 years ago and Abstract: The constantly growing bacterial resistance is being connected to antibiotic usage in clinical practice. against antibiotics is recently causing serious problems This clade can be distinguished by its increased number in the field of human and veterinary medicine as well as of mobile genetic elements, metabolic alternations and in agriculture. The mechanisms of resistance formation hypermutability [1]. All of these attributes led to the devel- and its preventions are not well explored in most bacterial opment of a flexible genome, which is now able to easily genera. The aim of this review is to analyse recent litera- adapt to the changes of the surroundings [2,3]. ture data on the principles of antibiotic resistance forma- It is also necessary to mention some basic informa- tion in bacteria of the Enterococcus genus. Furthermore, tion about morphological diversity, physiological and bio- the habitat of the Enterococcus genus, its pathogenicity chemical characteristics and taxonomy in order to under- and pathogenicity factors, its epidemiology, genetic and stand the resistance mechanisms completely. Biochemical molecular aspects of antibiotic resistance, and the rela- features must especially be mentioned since they play a tionship between these bacteria and bowel diseases are huge role in the high increase of resistance in these bac- discussed. -
Issn:0362-028X
Supplement A, 2017 Volume 80 Pages 2- CODEN: JFPRDR 79 (Sup)2-332 (2016) ISSN:0362-028X Supplement A, A, 2017 2017 Volume 80 80 Pages 1-332 2- CODEN: JFPRDR JFPRDR 80 79 (Sup)1-332 (Sup)2-332 (2017) (2016) ISSN: 0362-028X ISSN:0362-028X ABSTRACTS This is a collection of the abstracts from IAFP 2017, held in Tampa, Florida. 6200 Aurora Avenue, Suite 200W | Des Moines, Iowa 50322-2864, USA +1 800.369.6337 | +1 515.276.3344 | Fax +1 515.276.8655 www.foodprotection.org Journal of Food Protection Supplement 1 2 Journal of Food Protection Supplement Journal of Food Protection® ISSN 0362-028X Official Publication Reg. U.S. Pat. Off. Vol. 80 Supplement A 2017 Ivan Parkin Lecture Abstract ........................................................................................................................................... Ivan Parkin Lecture Abstract . 4 John H. Silliker Lecture Abstract .................................................................................................................................... John H . Silliker Lecture Abstract . 5 Abstracts Abstracts Special Symposium ................................................................................................................................................... SymposiumSymposium ................................................................................................................................................................. 7 RoundtableRoundtable .............................................................................................................................. -
Friedrich-Schiller-Universität Jena Biologisch-Pharmazeutische Fakultät Max-Planck-Institut Für Chemische Ökologie Lehrstuhl Für Bioorganische Chemie
Friedrich-Schiller-Universität Jena Biologisch-Pharmazeutische Fakultät Max-Planck-Institut für Chemische Ökologie Lehrstuhl für Bioorganische Chemie Control of gut microbiome by Lepidopteran pest Spodoptera littoralis Masterarbeit zur Erlangung des Grades eines Masters in Microbiology (M.Sc.) vorgelegt von Aishwarya Murali aus New Delhi (India) Jena, Januar 2019 Gutachter: 1. Prof. Dr.Wilhelm Boland, MPI for Chemical Ecology, Jena 2. Prof. Dr. Erika Kothe, Institute of Microbiology, FSU Jena 2 Confidential This master thesis work contains confidential data being prepared for ongoing publications. This work may only be available to the first and second examiners. Any publication and duplication of this master thesis – even in parts – is prohibited. 3 Table of Contents List of abbreviations…………………………………………………………………………6... List of figures…………………………………………………………………………………..9 List of tables…………………………………………………………………………………11… 1 Introduction…………………………………………………………………………….…12… 1.1 Basics of gut microbiota…………………………………………………………………….12 1.2 Insect gut microbiome…………………………………………………………………........13 1.3 Factors determining the gut community……………………………………………….........14 1.4 Lepidopteran insect hosts………………………………………………………………...15.... 1.5 Model organism: Spodoptera littoralis……………………………………………………..15 1.6 Predominant gut symbiont of S.littoralis: Enterococcus mundtii…………………………..18 1.7 Study of colonization and localization of E. mundtii by GFP based reporter method…...19... 1.8 Transformation of E. mundtii KD251……………………………………………………20… 1.9 Fluorescent Activated -
A Genomic View of Food-Related and Probiotic Enterococcus Strains
Dna Research, 2017, 24(1), 11–24 doi: 10.1093/dnares/dsw043 Advance Access Publication Date: 23 October 2016 Full Paper Full Paper A genomic view of food-related and probiotic Enterococcus strains Julieta Bonacina1, Nadia Suarez 1, Ricardo Hormigo1, Silvina Fadda1, Marcus Lechner2,*, and Lucila Saavedra1,* 1Laboratorio de Gene´tica y Biologıa Molecular, CERELA-CONICET, Centro de Referencia para Lactobacilos, San Miguel de Tucuman (T4000ILC), Tucuman, Argentina, and 2Department of Pharmaceutical Chemistry, Philipps- University Marburg, 35037 Marburg, Germany *To whom correspondence should be addressed. Tel. þ54 381 4310465 ext. 169. Fax: +54 381 4310465. ext 124. Email: [email protected] (L.S.); Tel. þ49 6421 2825925. Fax: +49 6421 2825854. Email: [email protected] (M.L.) Edited by Dr. Naotake Ogasawara Received 27 January 2016; Accepted 18 August 2016 Abstract The study of enterococcal genomes has grown considerably in recent years. While special attention is paid to comparative genomic analysis among clinical relevant isolates, in this study we performed an exhaustive comparative analysis of enterococcal genomes of food origin and/or with potential to be used as probiotics. Beyond common genetic features, we especially aimed to identify those that are specific to enterococcal strains isolated from a certain food-related source as well as features pre- sent in a species-specific manner. Thus, the genome sequences of 25 Enterococcus strains, from 7 different species, were examined and compared. Their phylogenetic relationship was reconstructed based on orthologous proteins and whole genomes. Likewise, markers associated with a successful colonization (bacteriocin genes and genomic islands) and genome plasticity (phages and clustered regularly interspaced short palindromic repeats) were investigated for lifestyle specific genetic fea- tures. -
Investigation Into the Influence of a Bacteriocin-Producing Enterococcus Strain on the Intestinal Microflora
Investigation into the influence of a bacteriocin-producing Enterococcus strain on the intestinal microflora Zur Erlangung des akademischen Grades eines Doktors der Naturwissenschaften an der Fakultät für Chemie und Biowissenschaften der Universität Karlsruhe genehmigte DISSERTATION von Agus Wijaya aus Palembang, Indonesien Referent: Prof. Dr. W.H. Holzapfel Co-Referent: Prof. Dr. W. Zumft Tag der mündlichen Prüfung: 02., 3., und 5. 12. 2003 ACKNOWLEDGMENT I would like to express my respect and appreciation to Prof. W.H. Holzapfel, Director of Institute of Hygiene and Toxicology in the Federal Research Centre for Nutrition (BFE), who has given me a recommendation needed in applying for DAAD (German Academic Exchange Service) scholarship. This recommendation has proven to be decisive in joint selection. I am very thankful for the chance he gave me to work in his excellent institute and for always being attentive when I needed his advice. Special thanks to Prof. Dr. W. Zumft for supporting my admission as Ph.D. student at the Fakultät für Chemie und Biowissenschaften, University of Karlsruhe and for his continued interest. My great gratitude comes to Dr. C.M.A.P. Franz, Head of the Molecular Microbiology Laboratory in BFE, for his dedicatory supervision and uncountable guidance throughout of my doctoral research. It would have been very difficult without his supervision and endless, valuable suggestions. I will always be grateful. I express my thank to Dr. Christian Neudecker who has given me valuable advice and guidance in preparing and implementing the animal experiment. My next round of thanks goes Mrs. Ingrid Specht and Ms. Anja Waldheim who has given me valuable technical assistance. -
The Gut Microbiota Composition of Trichoplusia Ni Is Altered by Diet and May Infuence Its Polyphagous Behavior M
www.nature.com/scientificreports OPEN The gut microbiota composition of Trichoplusia ni is altered by diet and may infuence its polyphagous behavior M. Leite‑Mondin1,4,5, M. J. DiLegge4,5, D. K. Manter2, T. L. Weir3, M. C. Silva‑Filho1 & J. M. Vivanco4* Insects are known plant pests, and some of them such as Trichoplusia ni feed on a variety of crops. In this study, Trichoplusia ni was fed distinct diets of leaves of Arabidopsis thaliana or Solanum lycopersicum as well as an artifcial diet. After four generations, the microbial composition of the insect gut was evaluated to determine if the diet infuenced the structure and function of the microbial communities. The population fed with A. thaliana had higher proportions of Shinella, Terribacillus and Propionibacterium, and these genera are known to have tolerance to glucosinolate activity, which is produced by A. thaliana to deter insects. The population fed with S. lycopersicum expressed increased relative abundances of the Agrobacterium and Rhizobium genera. These microbial members can degrade alkaloids, which are produced by S. lycopersicum. All fve of these genera were also present in the respective leaves of either A. thaliana or S. lycopersicum, suggesting that these microbes are acquired by the insects from the diet itself. This study describes a potential mechanism used by generalist insects to become habituated to their available diet based on acquisition of phytochemical degrading gut bacteria. Trichoplusia ni (Hübner, 1803) (Lepidoptera; Noctuidae) larvae are generalist herbivores and considered to be critical agricultural pests, which feed on more than 100 species of plants including tomato, potato, corn, cotton, and many other crops1.