Structure, Biomimetics, and Fluid Dynamics of Fish Skin Surfaces
Total Page:16
File Type:pdf, Size:1020Kb
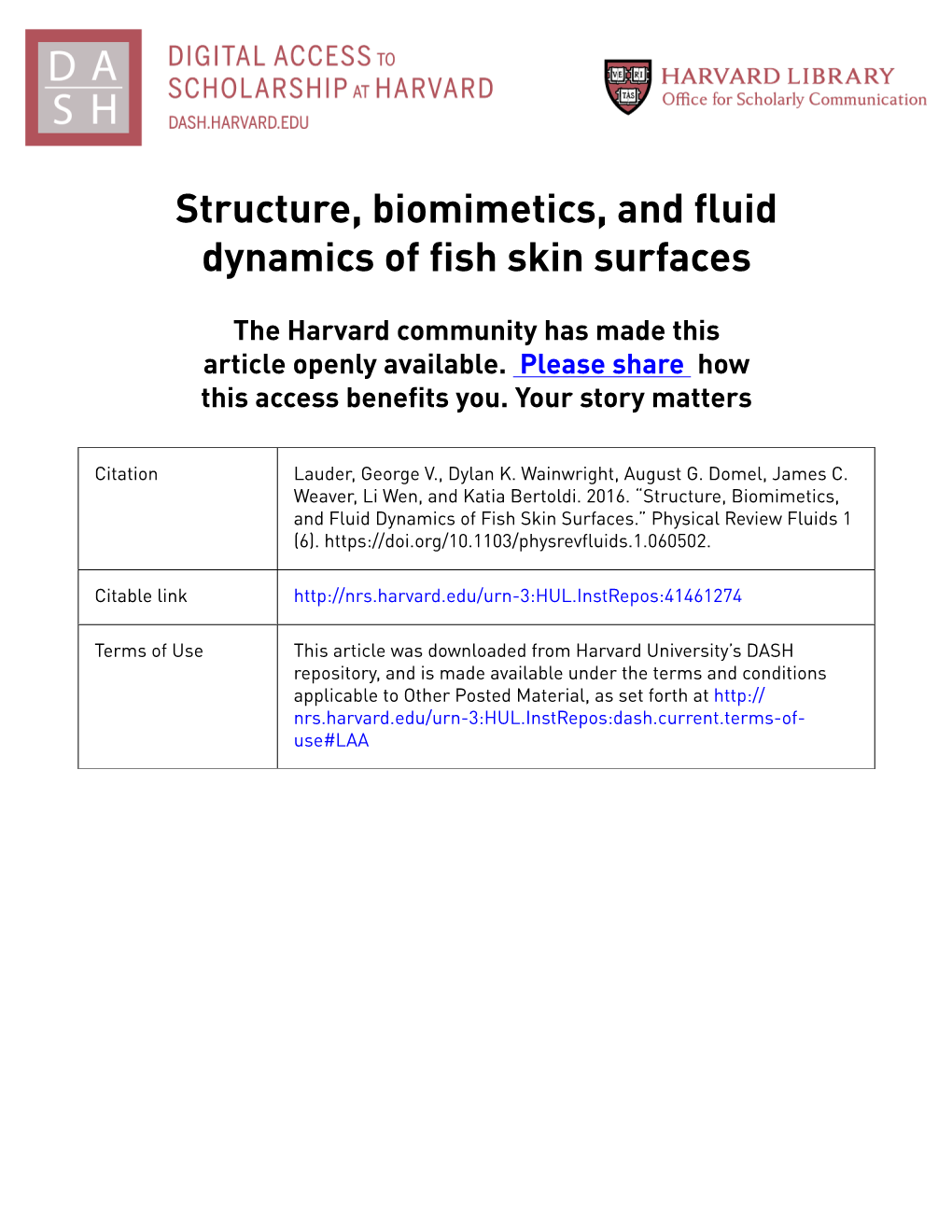
Load more
Recommended publications
-
Mechanics of Composite Elasmoid Fish Scale Assemblies and Their
journal of the mechanical behavior of biomedical materials 19 (2013) 75–86 Available online at www.sciencedirect.com www.elsevier.com/locate/jmbbm Mechanics of composite elasmoid fish scale assemblies and their bioinspired analogues Ashley Browninga, Christine Ortizb,n, Mary C. Boycea,n aDepartment of Mechanical Engineering, 77 Massachusetts Ave, Massachusetts Institute of Technology, Cambridge, MA, USA bDepartment of Materials Science and Engineering, 77 Massachusetts Ave, Massachusetts Institute of Technology, Cambridge, MA, USA article info abstract Article history: Inspired by the overlapping scales found on teleost fish, a new composite architecture Received 28 July 2012 explores the mechanics of materials to accommodate both flexibility and protection. These Received in revised form biological structures consist of overlapping mineralized plates embedded in a compliant 5 November 2012 tissue to form a natural flexible armor which protects underlying soft tissue and vital Accepted 11 November 2012 organs. Here, the functional performance of such armors is investigated, in which the Available online 24 November 2012 composition, spatial arrangement, and morphometry of the scales provide locally tailored Keywords: functionality. Fabricated macroscale prototypes and finite element based micromechanical Biomimetic models are employed to measure mechanical response to blunt and penetrating indentation Composite loading. Deformation mechanisms of scale bending, scale rotation, tissue shear, and tissue Natural armor constraint were found to govern the ability of the composite to protect the underlying Fish scale substrate. These deformation mechanisms, the resistance to deformation, and the resulting work of deformation can all be tailored by structural parameters including architectural arrangement (angle of the scales, degree of scale overlap), composition (volume fraction of the scales), morphometry (aspect ratio of the scales), and material properties (tissue modulus and scale modulus). -
Multi-Scale Analysis of Habitat Association in a Guild of Blennioid Fishes
MARINE ECOLOGY PROGRESS SERIES Vol. 125: 31-43.1995 Published September 14 Mar Ecol Prog Ser Multi-scale analysis of habitat association in a guild of blennioid fishes Craig Syms* University of Auckland Marine Laboratory, PO Box 349, Warkworth, New Zealand ABSTRACT: The degree to which reef fish are associated with parhcular reef characteristics has been the subject of much debate. It is increasingly clear that the strength of the relationship between reef fish and their habitat may be dependent on the scales at which the reef habitat is categorised. Conse- quently, scale must be explicitly incorporated into any investigation of fish and habitat association. I addressed the problem of scale by examining changes in the composition of a guild of blennioid fishes (comprising 13 species in the families Tripterygiidae and Blenniidae) relative to the scale at which their habitat was defined. Correspondence Analysis was used to display differences in guild structure At large, geographical scales, characteristic blenniold assemblages could be detected. Changes in gulld structure were due partly to differences in numerical dominance of a set of generalist species and, to a lesser extent, species composition. At broad scales, the blennioid assemblage displayed species- specific depth patterns and association with macroalgal cover. A core group of species was found at all depths, while others were restricted in depth and biogenic habitat type. The degree of shelter provided by topographic features characterised the blennioid assemblage at fine scales, and habitat specialisa- tion became apparent at this scale The patterns detected in this survey indicate scales at which ques- tions about processes generating these patterns may be profitably addressed. -
Scale Surface Structure of Mugil Cephalus (Teleostei; Mugilidae)
Biological Forum – An International Journal 7(1): 1845-1848(2015) ISSN No. (Print): 0975-1130 ISSN No. (Online): 2249-3239 Scale surface structure of Mugil cephalus (Teleostei; Mugilidae) using Scanning Electron Microscopy (SEM) Humera Zahid*, Nagina Bano*, Zubia Masood*, Musarrat Ul-Ain**, Rehana Yasmeen Farooq** and Wajeeha Razaq* *Department of Zoology, Sardar Bahadur Khan Women University Quetta, Balochistan, Pakistan. **Department of Zoology, University of Karachi, Karachi, Pakistan. (Corresponding author: Zubia Masood) (Received 17 April, 2015, Accepted 27 May, 2015) (Published by Research Trend, Website: www.researchtrend.net) ABSTRACT: As fish scale contain numerous microstructures that could be helpful for fish identification such as, ctenii, position of focus, circuli, annuli and radii. Therefore, in the present study, a traditional approach was made for studying in detail the structures of scales from a mullet species, Mugil cephalus (family Mugilidae). Samples were purchased from market of joint road, Quetta, Balochistan. During the study period extends from August 2014 to December 2014, total length of all collected specimens was ranged from 12.5- 17.5 cm, respectively. From each fish, scales were taken from the three different body regions i.e., HS (head scales), CS (caudal scales) and TRS (transverse row scales) in order to analyzed the variation in the microstructures on the scales. The scales have been subjected to scanning electron microscope (SEM) for the study of microstructures of scales in detail. Thus, from the result of the present study, it had been proved that in addition to external morphological characters of fish, some microstructures of scale could be helpful in systematic classification of any fish species. -
Training Manual Series No.15/2018
View metadata, citation and similar papers at core.ac.uk brought to you by CORE provided by CMFRI Digital Repository DBTR-H D Indian Council of Agricultural Research Ministry of Science and Technology Central Marine Fisheries Research Institute Department of Biotechnology CMFRI Training Manual Series No.15/2018 Training Manual In the frame work of the project: DBT sponsored Three Months National Training in Molecular Biology and Biotechnology for Fisheries Professionals 2015-18 Training Manual In the frame work of the project: DBT sponsored Three Months National Training in Molecular Biology and Biotechnology for Fisheries Professionals 2015-18 Training Manual This is a limited edition of the CMFRI Training Manual provided to participants of the “DBT sponsored Three Months National Training in Molecular Biology and Biotechnology for Fisheries Professionals” organized by the Marine Biotechnology Division of Central Marine Fisheries Research Institute (CMFRI), from 2nd February 2015 - 31st March 2018. Principal Investigator Dr. P. Vijayagopal Compiled & Edited by Dr. P. Vijayagopal Dr. Reynold Peter Assisted by Aditya Prabhakar Swetha Dhamodharan P V ISBN 978-93-82263-24-1 CMFRI Training Manual Series No.15/2018 Published by Dr A Gopalakrishnan Director, Central Marine Fisheries Research Institute (ICAR-CMFRI) Central Marine Fisheries Research Institute PB.No:1603, Ernakulam North P.O, Kochi-682018, India. 2 Foreword Central Marine Fisheries Research Institute (CMFRI), Kochi along with CIFE, Mumbai and CIFA, Bhubaneswar within the Indian Council of Agricultural Research (ICAR) and Department of Biotechnology of Government of India organized a series of training programs entitled “DBT sponsored Three Months National Training in Molecular Biology and Biotechnology for Fisheries Professionals”. -
18 Morphology of Scales in Three Teleost Species from Godavari River Basin in Parts of Maharashtra, India
International Journal of Zoology Studies International Journal of Zoology Studies ISSN: 2455-7269; Impact Factor: RJIF 5.14 www.zoologyjournals.com Volume 1; Issue 6; September 2016; Page No. 18-22 Morphology of scales in three teleost species from Godavari river basin in parts of Maharashtra, India Sumayya Ansari, * Shivaji Chavan, Sharda Padghane Fisheries Research Laboratory, Department of Zoology, School of Life Sciences, Swami Ramanand Teerth Marathwada University, Nanded, Maharashtra, India Abstract Scale surface morphology provides new and useful information on fish taxonomy. Several characters of scales are established and being used as a taxonomic tool. Scales in three teleosts species were observed and measured from eight regions of fish body that represented different size, shape and characters. From the results, it was concluded that scale morphology and its surface patterns can be valuable tools to investigate systematic relationship among the fish species, scale structure is also useful to recognize food chain in aquatic ecosystem which will be helpful to maintain and conserve different components in freshwater food chain. Keywords: Fish Scale, Morphology, Teleosts, Godavari river 1. Introduction the age of fish in years. The impressions and surface pattern of Fishes are one of the widely distributed and diverse group of circulii on scale served as a blue print for some physiological animals in the world. Due to overfishing & habitat destruction studies. Besides this there is role of scales in fish biology having this diversity is decreasing. Taxonomic identification of fishes numerous hidden details in their two or three dimensional design is essential to conserve them and to understand their role in the that helps effectively to identify and classify the fishes. -
Teleost Fish Scales Amongst the Toughest Collagenous Materials
journal of the mechanical behavior of biomedical materials 52 (2015) 95–107 Available online at www.sciencedirect.com www.elsevier.com/locate/jmbbm Research Paper Teleost fish scales amongst the toughest collagenous materials A. Khayer Dastjerdi, F. Barthelatn Department of Mechanical Engineering, McGill University, Montreal, QC, Canada article info abstract Article history: Fish scales from modern teleost fish are high-performance materials made of cross-plies of Received 27 May 2014 collagen type I fibrils reinforced with hydroxyapatite. Recent studies on this material have Received in revised form demonstrated the remarkable performance of this material in tension and against sharp 23 September 2014 puncture. Although it is known that teleost fish scales are extremely tough, actual Accepted 27 September 2014 measurements of fracture toughness have so far not been reported because it is simply Available online 6 October 2014 not possible to propagate a crack in this material using standard fracture testing fi Keywords: con gurations. Here we present a new fracture test setup where the scale is clamped Fish scales between two pairs of miniature steel plates. The plates transmit the load uniformly, Collagen prevent warping of the scale and ensure a controlled crack propagation. We report a À2 Toughness toughness of 15 to 18 kJ m (depending on the direction of crack propagation), which fi fi Process zone con rms teleost sh scales as one of the toughest biological material known. We also Crack bridging tested the individual bony layers, which we found was about four times less tough than the Bio-inspired materials collagen layer because of its higher mineralization. -
From Scales to Armour: Scale Losses and Trunk Bony Plate Gains in Ray
bioRxiv preprint doi: https://doi.org/10.1101/2020.09.09.288886; this version posted September 9, 2020. The copyright holder for this preprint (which was not certified by peer review) is the author/funder, who has granted bioRxiv a license to display the preprint in perpetuity. It is made available under aCC-BY-NC 4.0 International license. 1 From scales to armour: scale losses and trunk bony plate gains in ray- 2 finned fishes 3 Alexandre Lemopoulos1, Juan I. Montoya-Burgos1,2,3 4 1. Department of Genetics and Evolution. University of Geneva, Geneva, Switzerland 5 2. iGE3 institute of Genetics and Genomics of Geneva 6 3. E-mail: [email protected] ORCID: https://orcid.org/0000-0001-9080-9820 7 Article type : Letter 8 Running title: Tegument cover transitions along actinopterygian evolution 9 Keywords: Tegument, actinopterygians, gene network, skeleton evolution, functional 10 innovation, ancestral state, phylogeny 11 12 Abstract 13 Actinopterygians (ray-finned fishes) are the most diversified group of vertebrates and are 14 characterized by a variety of protective structures covering their tegument, the evolution of 15 which has intrigued biologists for decades. Paleontological records showed that the first 16 mineralized vertebrate skeleton was composed of dermal bony plates covering the body, 17 including odontogenic and skeletogenic components. Later in evolution, the exoskeleton of 18 actinopterygian's trunk was composed of scale structures. Although scales are nowadays a 19 widespread tegument cover, some contemporary lineages do not have scales but bony 20 plates covering their trunk, whereas other lineages are devoid of any such structures. -
Investigation of the Inherent Chemical, Structural, and Mechanical Attributes of Bio-Engineered Composites Found in Nature
University of Arkansas, Fayetteville ScholarWorks@UARK Theses and Dissertations 12-2015 Investigation of the Inherent Chemical, Structural, and Mechanical Attributes of Bio-Engineered Composites Found in Nature: Alligator Gar’s Exoskeleton Fish Scales Wayne Derald Hodo University of Arkansas, Fayetteville Follow this and additional works at: http://scholarworks.uark.edu/etd Part of the Biochemistry Commons, Biomechanics and Biotransport Commons, and the Structural Biology Commons Recommended Citation Hodo, Wayne Derald, "Investigation of the Inherent Chemical, Structural, and Mechanical Attributes of Bio-Engineered Composites Found in Nature: Alligator Gar’s Exoskeleton Fish Scales" (2015). Theses and Dissertations. 1425. http://scholarworks.uark.edu/etd/1425 This Dissertation is brought to you for free and open access by ScholarWorks@UARK. It has been accepted for inclusion in Theses and Dissertations by an authorized administrator of ScholarWorks@UARK. For more information, please contact [email protected], [email protected]. Investigation of the Inherent Chemical, Structural, and Mechanical Attributes of Bio-Engineered Composites Found in Nature: Alligator Gar’s Exoskeleton Fish Scales A dissertation submitted in partial fulfillment of the requirements for the degree of Doctor of Philosophy in Engineering by Wayne D. Hodo Auburn University Bachelor of Science, 1997 Mississippi State University Master of Science in Civil Engineering, 2006 December 2015 University of Arkansas This dissertation is approved for recommendation to the Graduate -
Fish SD: Tale of a Scale
Fish SD: Tale of a Scale Background: There are four types of fish scales - Background information: Review placoid, cycloid, ctenoid (pronounced ‘ten-oid’), and Chapter 6: Fish Anatomy and 9: ganoid. Most bony fish have cycloid scales. Fish with Fisheries Management in Going cycloid scales have the same number of scales their Fishing entire lives - the scales enlarge to accommodate a Duration: 45 minutes fish’s growth (scales that are lost to injury will be regrown). This results in a pattern of concentric Materials: Copies of Crappie Scale growth rings on the scale, which look similar to the Impression Worksheet; markers, growth rings in the trunk of a tree. The growth rings colored pencils or crayons; on a scale are known by scientists as circuli (singular transparency of fish scale to lead class circulus). Objectives: Students will correlate different seasons with a fish’s growth. Just like counting the rings of a tree, biologists can determine the age of a fish by reading its scales with a microscope. How do biologists do this? Actually, aging fish is fairly easy. The development of circuli is similar to that of tree rings. During periods of rapid growth, the rings are widely spaced and when growth slows, the rings are constricted together. Rings are formed on a cycloid scale constantly, so biologists can read seasons of growth. In the Great Plains, fish will grow rapidly in the summer months when water temperatures and food availability are highest; they experience slower growth during the colder winter months. Therefore, the scales typically exhibit bands of widely spaced growth rings (summer) separated by constrictions (winter). -
A Genetic Analysis of Cichlid Scale Morphology
University of Massachusetts Amherst ScholarWorks@UMass Amherst Masters Theses Dissertations and Theses November 2016 A Genetic Analysis of Cichlid Scale Morphology Kenta C. Kawasaki University of Massachusetts Amherst Follow this and additional works at: https://scholarworks.umass.edu/masters_theses_2 Part of the Developmental Biology Commons, and the Genetics Commons Recommended Citation Kawasaki, Kenta C., "A Genetic Analysis of Cichlid Scale Morphology" (2016). Masters Theses. 425. https://doi.org/10.7275/9004629 https://scholarworks.umass.edu/masters_theses_2/425 This Open Access Thesis is brought to you for free and open access by the Dissertations and Theses at ScholarWorks@UMass Amherst. It has been accepted for inclusion in Masters Theses by an authorized administrator of ScholarWorks@UMass Amherst. For more information, please contact [email protected]. A GENETIC ANALYSIS OF CICHLID SCALE MORPHOLOGY A Thesis Presented by KENTA C. KAWASAKI Submitted to the Graduate School of the University of Massachusetts Amherst in partial fulfillment of the requirements for the degree of MASTER OF SCIENCE September 2016 Molecular and Cellular Biology © Copyright by Kenta C. Kawasaki 2016 All Rights Reserved A GENETIC ANALYSIS OF CICHLID SCALE MORPHOLOGY A Thesis Presented by KENTA C. KAWASAKI Approved as to style and content by: _________________________________ Craig Albertson, Chair _________________________________ Courtney Babbitt, Member _________________________________ Hélène Cousin, Member _______________________________ Dominique Alfandari, Program Leader / GPD, Molecular and Cellular Biology Program ABSTRACT A GENETIC ANALYSIS OF CICHLID SCALE MORPHOLOGY SEPTEMBER 2016 KENTA C. KAWASAKI, B.S., UNIVERSITY OF MASSACHUSETTS AMHERST M.S., UNIVERSITY OF MASSACHUSETTS AMHERST Directed by: Professor Craig Albertson Epidermal appendages are found on every vertebrate this world has to offer. -
For Coral Reef Fishes Chaetodontidae Family
769 International Journal of Progressive Sciences and Technologies (IJPSAT) ISSN: 2509-0119. © 2019 International Journals of Sciences and High Technologies http://ijpsat.ijsht-journals.org Vol. 17 No. 2 November 2019, pp. 110-116 Three Point Sampling Scales Chaetodontidae Method (TPSSCM) For Coral Reef Fishes Chaetodontidae Family Abdul Razak1* and Vauzia2 1Associate Professor at Departement Biology, Universitas Negeri Padang 2Associate Professor at Departement Biology, Universitas Negeri Padang Abstract - Coral reef fishes Chaetodontidae family have characteristic scales and linea lateralis position. This coral reef fishes family unique and have some color and specific as ornamental salt water fishes. This article aims to describe scales different point: dorsal point, linea lateralis point and ventral point are different. The scales sampling taken according Three Point Sampling Scales Chaetodontidae Method (TPSSCM). They are as reasons for new technic sampling. Until now, no research about scales of Coral Reef Fishes Chaetodontidae.The result of the research showed microstructure scales dorsal point, linea lateralis point and ventral point are different. Different component scales at dorsal point, linea lateralis point and ventral point are indicate scales of Coral Reef Fishes Chaetodontidae specific and unique if we are going compare to another scales fishes consumption or scales of freshwater fishes. Conclusion, Three Point Sampling Scales Chaetodontidae Method (TPSSCM) can indicated different position of scales are different morphology or shape of scale elements. Keywords - Coral reef fishes, Chaetodontidae, scale, Three Point Sampling Scales Chaetodontidae Method (TPSSCM). I. INTRODUCTION appearance of the lateral, anterior, and posterior fields; and to some extent, thickness/robustness of the scale. As there is One of the unique features in a fish is the presence of the considerable variation in scale shape even between different scales. -
Structure and Mechanical Properties of Selected Protective Systems in Marine Organisms
UC San Diego UC San Diego Previously Published Works Title Structure and mechanical properties of selected protective systems in marine organisms. Permalink https://escholarship.org/uc/item/5dv5w5ww Authors Naleway, Steven E Taylor, Jennifer RA Porter, Michael M et al. Publication Date 2016-02-01 DOI 10.1016/j.msec.2015.10.033 License https://creativecommons.org/licenses/by-nc/4.0/ 4.0 Peer reviewed eScholarship.org Powered by the California Digital Library University of California Materials Science and Engineering C 59 (2016) 1143–1167 Contents lists available at ScienceDirect Materials Science and Engineering C journal homepage: www.elsevier.com/locate/msec Review Structure and mechanical properties of selected protective systems in marine organisms Steven E. Naleway a,⁎,JenniferR.A.Taylorb, Michael M. Porter e, Marc A. Meyers a,c,d, Joanna McKittrick a,c a Materials Science and Engineering Program, University of California, San Diego, La Jolla, CA 92093, USA b Scripps Institution of Oceanography, University of California, San Diego, La Jolla, CA 92037, USA c Department of Mechanical and Aerospace Engineering, University of California, San Diego, La Jolla, CA 92093, USA d Department of NanoEngineering, University of California, San Diego, La Jolla, CA 92093, USA e Department of Mechanical Engineering, Clemson University, Clemson, SC 29634, USA article info abstract Article history: Marine organisms have developed a wide variety of protective strategies to thrive in their native environments. Received 21 January 2015 These biological materials, although formed from simple biopolymer and biomineral constituents, take on many in- Received in revised form 29 September 2015 tricate and effective designs.