300 Area Treatability Test: Laboratory Development of Polyphosphate
Total Page:16
File Type:pdf, Size:1020Kb
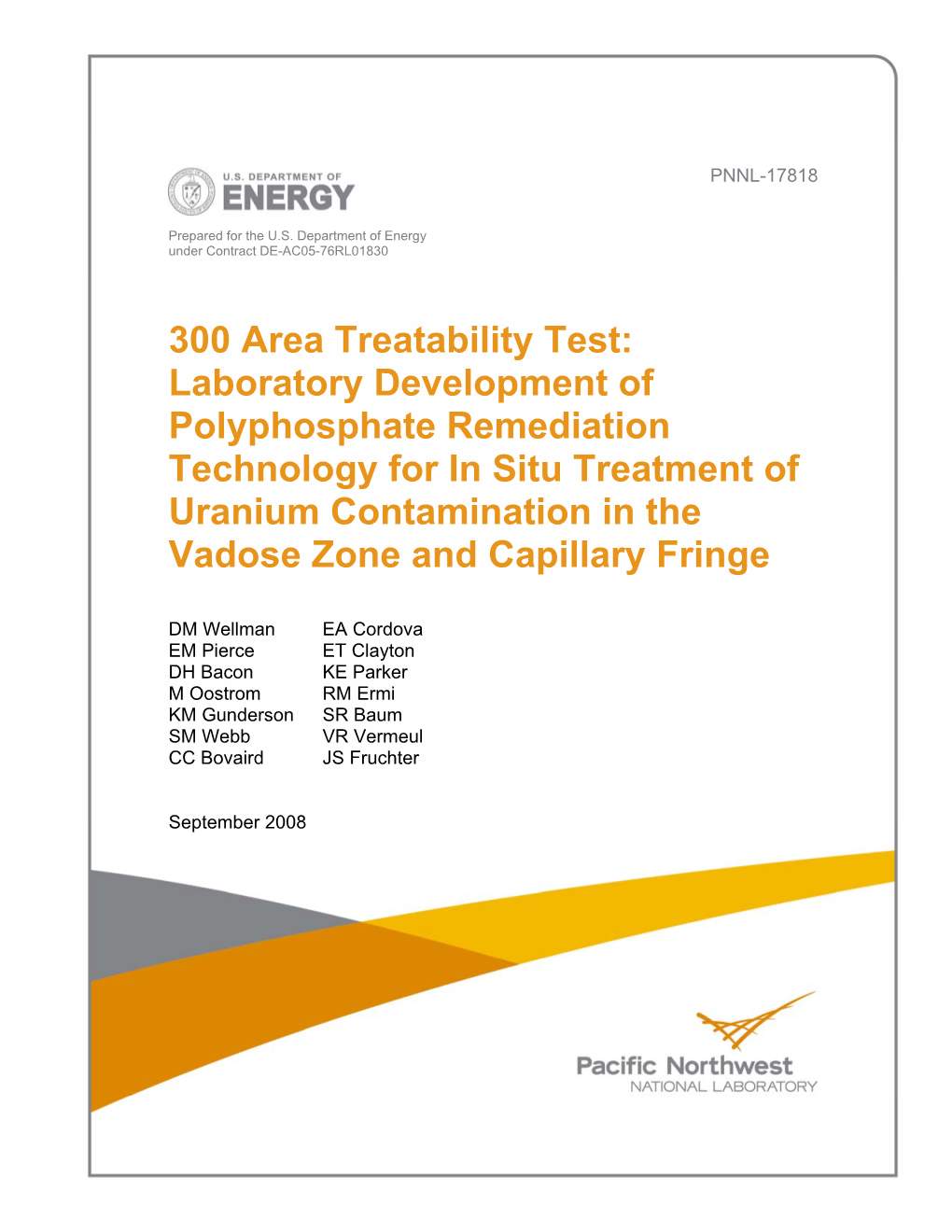
Load more
Recommended publications
-
Remediation of Uranium-Contaminated Ground Water at Fry Canyon, Utah
Science Highlight – November 2003 Remediation of Uranium-contaminated Ground Water at Fry Canyon, Utah Christopher C. Fuller1, John R. Bargar2, James A. Davis1 1U.S. Geological Survey, Water Resources Division, Menlo Park, CA 2Stanford Synchrotron Radiation Laboratory, SLAC, Stanford University, Stanford, CA Clean up of contaminated aquifers is a difficult and expensive problem because of the inaccessibility of the subsurface and the volume of soil and ground water requiring treat- ment. Established technologies such as pump-and-treat and soil excavation are ineffective in most large contamination scenarios because they treat only a small fraction of the con- tamination or are prohibitively expensive (National Research Council, 1999). Perme- able Reactive Barriers (PRBs) are a relatively new technology that offer promise to overcome these obstacles. PRBs are trenches (figure 1) or fence-like arrays of non-pumping wells emplaced in the subsurface at depths of up to 150 feet to intercept the flow of contaminated ground water (Freethey et al., 2002). Fill materials contained within the PRBs react with dissolved contaminants to degrade or sequester them. Thus, in essence, PRBs act as large in-situ filters to clean ground water. PRB technologies offer lower oper- ating costs, are highly energy efficient, and require no surface facilities or ground water pumping/recharge (Freethey et al., 2002; Morrison and Spangler, 1992; Shoemaker et al., 1995). Two commonly proposed PRB contaminant-removal mechanisms are: (a) precipitation reac- tions in which metal contaminants are sequestered within freshly formed mineral phases, and (b) oxidative degradation of contaminants by particulate iron metal. In order for PRBs to be cost-effective they should be effective for an economically viable period (or be replenishable). -
Iidentilica2tion and Occurrence of Uranium and Vanadium Identification and Occurrence of Uranium and Vanadium Minerals from the Colorado Plateaus
IIdentilica2tion and occurrence of uranium and Vanadium Identification and Occurrence of Uranium and Vanadium Minerals From the Colorado Plateaus c By A. D. WEEKS and M. E. THOMPSON A CONTRIBUTION TO THE GEOLOGY OF URANIUM GEOLOGICAL S U R V E Y BULL E TIN 1009-B For jeld geologists and others having few laboratory facilities.- This report concerns work done on behalf of the U. S. Atomic Energy Commission and is published with the permission of the Commission. UNITED STATES GOVERNMENT PRINTING OFFICE, WASHINGTON : 1954 UNITED STATES DEPARTMENT OF THE- INTERIOR FRED A. SEATON, Secretary GEOLOGICAL SURVEY Thomas B. Nolan. Director Reprint, 1957 For sale by the Superintendent of Documents, U. S. Government Printing Ofice Washington 25, D. C. - Price 25 cents (paper cover) CONTENTS Page 13 13 13 14 14 14 15 15 15 15 16 16 17 17 17 18 18 19 20 21 21 22 23 24 25 25 26 27 28 29 29 30 30 31 32 33 33 34 35 36 37 38 39 , 40 41 42 42 1v CONTENTS Page 46 47 48 49 50 50 51 52 53 54 54 55 56 56 57 58 58 59 62 TABLES TABLE1. Optical properties of uranium minerals ______________________ 44 2. List of mine and mining district names showing county and State________________________________________---------- 60 IDENTIFICATION AND OCCURRENCE OF URANIUM AND VANADIUM MINERALS FROM THE COLORADO PLATEAUS By A. D. WEEKSand M. E. THOMPSON ABSTRACT This report, designed to make available to field geologists and others informa- tion obtained in recent investigations by the Geological Survey on identification and occurrence of uranium minerals of the Colorado Plateaus, contains descrip- tions of the physical properties, X-ray data, and in some instances results of chem- ical and spectrographic analysis of 48 uranium arid vanadium minerals. -
AUTUNITE from MT. SPOKANE, WASHINGTON* G. W. Lno, U. S. Geologicalsurvey, Menlo Park, California
THE AMERICAN MINERALOGIST, VOL. 45, JANUARY_FEBRUARY, 1960 AUTUNITE FROM MT. SPOKANE, WASHINGTON* G. W. Lno, U. S. GeologicalSurvey, Menlo Park, California ABSTRACT Near Mt. Spokane, Washington, coarsely crystalline autunite is developed in vugs, fractures, and shear zones in granitic rock. With the exception of dispersed submicroscopic uraninite particles, autunite is the only ore mineral in the deposits. A study of associated granitic rocks reveals that apatite, the most abundant accessory constituent, has been pref- erentially leached and corroded in mineralized zones, suggesting that it may have provided a source of lime and phosphate for the formation of autunite. Leaching may have been effected partly by meteoric water) but more probably was due to the action of ascending connate solutions that may also have carried uranium from unoxidized, as yet undiscovered deposits at depth. Autunite from the Daybreak mine has been studied optically, chemically, and by r-ray diffraction. The autunite is commonly zoned from light-yellow margins to dark-green or black cores, and autunite from the inner zone has a higher specific gravity and higher re- fractive indices than peripheral light material. X-ray powder difiraction patterns of dark and light meta-autunite formed from this autunite show no significant difierences in the d spacings; howevet, diffraction patterns of nine zoned samples each show uraninite to be present in the dark, and absent from the light, phase. UOz and UOs determinations range from 0.66-0.70 per cent and 57.9-58.0 pe( cent, respectively, for light autunite, whereas dark autunite shows a range (in seven determinations) of UOz from 1,2 to 4.0 per cent, and UOs from 55.1 to 58.8 per cent. -
Cation Substitution in Uranyl Phosphates of the Autunite Group: Equilibrium Relations and Crystallization Between Metatorbernite and Metauranocircite
Versão online: http://www.lneg.pt/iedt/unidades/16/paginas/26/30/208 Comunicações Geológicas (2015) 102, Especial I, 27-30 ISSN: 0873-948X; e-ISSN: 1647-581X Cation substitution in uranyl phosphates of the autunite group: equilibrium relations and crystallization between metatorbernite and metauranocircite Substituição catiónica em fosfatos de uranilo do grupo da autunite: relações de equilíbrio e cristalização entre metatorbernite e metauranocircite M. Andrade1, J. Duarte1, I. Martins 1, J. Reis 1, J. Mirão3, M. A. Gonçalves1,2* Artigo original Original article © 2015 LNEG – Laboratório Nacional de Geologia e Energia IP Abstract: Uranyl phosphate minerals play an important role in the 1. Introduction uranium immobilization within weathering and supergene enrichment profiles. This work consists on the morphological, structural and Uranyl phosphate minerals are major constituents in weathered U chemical characterization of natural and synthetic minerals of Cu and Ba deposits and can display a multi-stage evolving history in the – metatorbernite and metauranocircite, respectively. SEM imaging has environment they crystalize. Their importance is two-fold: as revealed an extended range of morphologies, from tabular to rosette-like main U-bearing phases in weathering profiles with potential crystals, with the presence of epitaxial growths. These studies have also economic value (as in Nisa and Tarabau, where natural uranyl revealed natural heterogeneities affected by cationic substitution along phosphates of Cu and Ba were identified; Pinto et al., 2012; preferred crystallographic directions. The experimental results suggest Prazeres, 2011) and as fixing phases of U limiting its long-term, that the precipitation of metatorbernite is easier than metauranocircite. Simulations of the chemical system show that precipitation depends on million-year scale, dispersion in the oxidized surface supersaturation evolution, which in turn in a function of aqueous complex environment. -
Spodumene and Autunite from Alstead, New Hampshire G
578 TEE AM ERICAN M I N ERALOGIST SPODUMENE AND AUTUNITE FROM ALSTEAD, NEW HAMPSHIRE G. R. MBcarHLrN, Cornel'|,Uniaersity. During an investigation in August and September, 7927, oI the pegmatites of the Gilsum area, Cheshire County, New Hamp- shire, two minerals, spodumene and autunite, were found which are not known to have been reported before from this district. It seemsworthwhile, therefore, to put this occurrence on record. The pegmatites of this region are intruded into a mica schist with which they have rather sharp contacts, although there is some lit-par-lit injection on a small scale as well as tourmalini- zation of the wall rock. The essentialminerals, aside from qtartz, are feldspar and muscovite, both of which are extensively quarried in the area. The accessory minerals include biotite, black tour- maline, beryl, garnet, apatite, spodumene,autunite, and zircon. The spodumene and autunite came from the quarry of the New Hampshire Mica and Mining Company in the town of Alstead, about one and four-fifths miles north northwest of Gilsum village. The spodumene crystals occur on an inaccessiblewall near the top of the west face of the quarry. They were much concealedby material washed down from the surface, but the crystals exposed appeared to be of the order of several feet in length. From blocks which had fallen from the wall a few crystals were obtained, the largest of which was six inches long, four inches wide, and one and one-half inches thick, but it did not represent the entire crystal. The spodumene occurs associated with tourmaline, garnet, apatite, feldspar, qtartz, and sericite. -
Meta-Autunite Ca(UO2)2(PO4)2 • 2−6H2O C 2001-2005 Mineral Data Publishing, Version 1
Meta-autunite Ca(UO2)2(PO4)2 • 2−6H2O c 2001-2005 Mineral Data Publishing, version 1 Crystal Data: Tetragonal. Point Group: 4/m 2/m 2/m or 422. As rectangular tabular crystals, exhibiting {001}, {111}, {110}, {011}, rarely pyramidal; as foliated or scaly aggregates. Physical Properties: Cleavage: Perfect on {001}; indistinct on {010}. Hardness = 2–2.5 D(meas.) = 3.35–3.55 D(calc.) = 3.31 Radioactive. Fluoresces yellowish green under UV. Dehydrates irreversibly from autunite under ambient conditions. Optical Properties: Translucent to opaque. Color: Lemon-yellow to greenish yellow, yellowish green, dark green. Luster: Pearly to dull. Optical Class: Uniaxial (–), anomalously biaxial (–). Pleochroism: X = colorless to pale yellow; Y = Z = dark yellow. Orientation: Z = c. Dispersion: r> v,strong. ω = 1.600–1.611 = 1.594–1.598 α = 1.596–1.604 β = 1.602–1.622 γ = 1.603–1.630 2V(meas.) = 5–20◦ Cell Data: Space Group: P 4/nmm or P 4222. a = 6.99 c = 8.46 Z = 1 X-ray Powder Pattern: Les Oudots mine, Saˆone-et-Loire,France. (ICDD 39-1351). 8.46 (100), 3.620 (60), 2.115 (35), 2.615 (30), 1.601 (25), 5.39 (20), 4.233 (20) Chemistry: (1) The mineral usually analyzed as autunite; meta-autunite I contains 6H2O; meta-autunite II contains 2H2O and probably does not occur in nature. Mineral Group: Meta-autunite group. Occurrence: A secondary mineral, formed as dehydration pseudomorphs after autunite. Association: Autunite. Distribution: Widespread. All autunite (q.v.) localities probably contain meta-autunite. Name: The prefix meta indicates the dehydration product of autunite. -
Diagenetic Formation of Uranium-Silica Polymers in Lake Sediments Over 3,300 Years
Diagenetic formation of uranium-silica polymers in lake sediments over 3,300 years Pierre Lefebvrea,1, Alkiviadis Gourgiotisb, Arnaud Mangeretb, Pierre Sabatierc, Pierre Le Papea, Olivier Diezb, Pascale Louvatd, Nicolas Menguya, Pauline Merrota,2, Camille Bayaa, Mathilde Zebrackib, Pascale Blanchartb, Emmanuel Maletc, Didier Jézéqueld,e, Jean-Louis Reyssc, John R. Bargarf, Jérôme Gaillardetd, Charlotte Cazalab, and Guillaume Morina aInstitut de Minéralogie, de Physique des Matériaux et de Cosmochimie, UMR 7590 Sorbonne Université-CNRS-MNHN-IRD (Museum National d’Histoire Naturelle-Institut de Recherche pour le Développement), 75005 Paris, France; bInstitut de Radioprotection et de Sûreté Nucléaire, PSE-ENV SEDRE, 92260 Fontenay-aux-Roses, France; cUMR 5204, Université Grenoble Alpes-Université Savoie Mont Blanc-CNRS-EDYTEM (Environnements DYnamiques et TErritoires de la Montagne), 73370 Le Bourget-Du-Lac, France; dUMR 7154, Université de Paris-Institut de Physique du Globe de Paris-CNRS, 75005 Paris, France; eINRAE-Université Savoie Mont Blanc, UMR CARRTEL (Centre Alpin de Recherche sur les Réseaux Trophiques et Ecosystèmes Limniques), 74200 Thonon-les-Bains, France; and fStanford Synchrotron Radiation Lightsource, SLAC National Accelerator Laboratory, Menlo Park, CA 94025 Edited by François M. M. Morel, Princeton University, Princeton, NJ, and approved December 16, 2020 (received for review October 27, 2020) The long-term fate of uranium-contaminated sediments, especially have been recognized as a major U pool in reduced contami- downstream former mining areas, is a widespread environmental nated soils and sediments over the last decade (6, 7, 19–25) and challenge. Essential for their management is the proper under- are known to be sensitive to reoxidation by natural oxidants such standing of uranium (U) immobilization mechanisms in reducing as oxygen or nitrate (26 and references therein). -
Download the Scanned
American Mineralogist, Volume74, pages 1399-1404, 1989 NEW MINERAL NAMES* JonN L. Jamnon CANMET, 555 Booth Street,Ottawa, Ontario KIA OGl, Canada EnNsr A. J. Bunxe Instituut voor Aardwetenschappen,Vrije Universiteite, De Boelelaan 1085, l08l HV, Amsterdam, Netherlands Blatteritex 814-940 (average877). In reflectedlight, slightly to mod- eratelybireflectant, nonpleochroic; variation in color (buff G. Raade,M.H. Mladeck, V.K. Din, A.J. Criddle, C.J. weak to Stanley(1988) Blatterite, a new Sb-bearingMn2+-Mn3+ to pale bufl) is due to bireflectance.Anisotropy member of the pinakiolite group, from Nordmark, distinct, with rotation tints in shadesof grayish-brown. Sweden.Neues Jahrb. Mineral. Mon., l2l-136. No twinning. Orange-redinternal reflections.Reflectance data aregiven at intervals of l0 nm from 400 to 700 nm The empirical formula was calculatedfrom an analysis in air and oil. Reflectanceis about 110/oin air. X, Y, and rce of 2.53 mg of hand-picked by emissionspectrometry Z axescorrespond to a, c, and Daxes, with the optic plane fragments.Recalculation to conform with the gen- crystal parallel to (001). The sign ofbireflectance in air changes eral formula of the pinakiolite group yielded a MnO- from positive (400-450 nm) to negative (470-700 nm). MnrO, distribution, confirmed by a wet-chemical analy- The sign of birefringenceis positive from 400 to 520 nm, sis on 960 pg of material.The result is MgO 13.0,FerO, and negative from 520 to 700 nm. Dispersion r < v. 3.48,MnO 35.1,MnrOr 22.2,SbrO3 11.4, B2O3 14.4,to- Color valuesare also given. -
Uranophane Ca(UO2)2(Sio3oh)2 ² 5H2O C 2001 Mineral Data Publishing, Version 1.2 ° Crystal Data: Monoclinic
Uranophane Ca(UO2)2(SiO3OH)2 ² 5H2O c 2001 Mineral Data Publishing, version 1.2 ° Crystal Data: Monoclinic. Point Group: 2: Crystals are composite needles, elongated [010], to over 1 cm, poorly developed, warped or bent. As stellate aggregates; in ¯brous or felted k crusts, and massive. Physical Properties: Cleavage: Perfect on 100 (?); traces of another, parallel elongation. Tenacity: Brittle. Hardness = 2.5 D(meas.)f= 3.g81{3.90 D(calc.) = 3.78 Crystals weakly » °uorescent green under UV, typically not °uorescent when massive; radioactive. Optical Properties: Transparent to translucent. Color: Lemon-yellow to pale straw-yellow or honey-brown; also greenish yellow to yellowish green, yellow-orange. Luster: Vitreous, pearly on the good cleavage; waxy or dull when massive. Optical Class: Biaxial ({). Pleochroism: Weak; X = colorless; Y = pale canary-yellow; Z = canary-yellow. Orientation: Z = b; X 100 cleavage or a °at face. Dispersion: r < v; strong, ? f g crossed. ® = 1.642{1.648 ¯ = 1.661{1.667 ° = 1.667{1.675 2V(meas.) = 32±{49± Cell Data: Space Group: P 21: a = 15.858{15.909 b = 6.985{7.002 c = 6.641{6.665 ¯ = 97 27 97 33 Z = 2 ± 0¡ ± 0 X-ray Powder Pattern: Ruggles pegmatite, Grafton Co., New Hampshire, USA. 7.88 (100), 3.94 (90), 2.99 (80), 2.91 (80), 1.969 (70), 4.76 (50), 3.20 (50) Chemistry: (1) (2) SiO2 12.66 14.03 UO3 65.24 66.80 CaO 8.53 6.55 H2O 13.02 12.62 Total 99.45 100.00 (1) Lusk, Wyoming, USA. -
Thermodynamic Properties of Autunite, Uranyl Hydrogen Phosphate, And
Environ. Sci. Technol. 2009, 43, 7416–7422 phosphate application represents a promising remediation Thermodynamic Properties of technology for uranium contamination of groundwater Autunite, Uranyl Hydrogen aquifers. In this approach, focused application of polyphos- phate can reduce the concentration of aqueous uranium Phosphate, and Uranyl through direct precipitation of uranyl phosphate minerals (1). Modeling and optimization of this approach requires Orthophosphate from Solubility and knowledge of thermodynamic properties for the range of uranyl phosphate mineral phases that can form under realistic Calorimetric Measurements environmental conditions. Although the thermodynamic properties of some environmentally relevant uranyl phos- DREW GORMAN-LEWIS,*,† phate phases have been measured (e.g., see ref 2 for a recent TATIANA SHVAREVA,‡ review of the available solubility data), the data set lacks KARRIE-ANN KUBATKO,†,# some crucial phases, and the thermodynamic properties for PETER C. BURNS,† DAWN M. WELLMAN,§ some of the uranyl phosphate phases that have been studied BRUCE MCNAMARA,§ are poorly constrained. JENNIFER E. S. SZYMANOWSKI,† In this study, we measured the solubilities of uranyl ‡ ALEXANDRA NAVROTSKY, AND hydrogen phosphate (UO2HPO4 · 3H2O) (abbreviated as HUP), † JEREMY B. FEIN uranyl orthophosphate ((UO2)3(PO4)2 · 4H2O) (UP), and au- Department of Civil Engineering and Geological Sciences, tunite (Ca[(UO2)(PO4)]2 · 3H2O) (CaUP), using the results to University of Notre Dame, 156 Fitzpatrick Hall, Notre Dame, determine the Gibbs free energies of formation of each phase. Indiana 46556, Peter A. Rock Thermochemistry Laboratory, In addition, we used high-temperature oxide melt solution University of California Davis, One Shields Avenue, Davis, calorimetry to yield the enthalpies of formation of HUP and California 95616, and Pacific Northwest National Laboratory, UP. -
Metatorbernite Cu(UO2)2(Aso4)2·8H2O
Metatorbernite Cu(UO2)2(AsO4)2·8H2O Crystal Data: Tetragonal. Point Group: 4/m. Typically pseudomorphous after torbernite by stepped dehydration, as square tabular crystals, flattened on {001} and modified by {011}, in lamellar or subparallel to sheaflike aggregates, and as rosettes, to 2 cm. Physical Properties: Cleavage: Perfect on {001}; indistinct on {010}. Tenacity: Brittle. Hardness = 2.5 D(meas.) = 3.52-3.70 D(calc.) = 3.689 Radioactive. Optical Properties: Transparent to translucent. Color: Pale green to dark green. Luster: Vitreous, subadamantine, pearly on {001}. Optical Class: Uniaxial (+) or uniaxial (–); anomalously biaxial in sectors. ω = 1.618-1.631 ε = 1.622-1.628 Pleochroism: Weak; O = green, E = pale green to blue. Absorption: O > E. Dispersion: r > v, extreme. Cell Data: Space Group: P4/n. a = 6.9756(5) c = 17.349(2) Z = 2 X-ray Powder Pattern: Schneeberg, Germany. 8.71 (100), 3.678 (100), 3.480 (80), 3.232 (80), 5.44 (75), 4.93 (75), 2.931 (70) Chemistry: (1) (2) UO3 59.67 61.01 P2O5 14.00 15.14 SiO2 0.40 CuO 8.50 8.48 H2O 15.00 15.37 Total 97.57 100.00 (1) Gunnislake mine, England. (2) Cu(UO2)2(PO4)2∙8H2O. Mineral Group: Meta-autunite group. Occurrence: Typically a secondary mineral, a dehydration product of torbernite formed during weathering; formed directly above 75 °C. Association: Torbernite, meta-autunite. Distribution: Widespread; probably occurs at all localities for torbernite (q.v.). First described from Schneeberg, Saxony, Germany. Material from the Gunnislake mine, Calstock, Cornwall, England, is thought to be primary. -
Autunite-Group Minerals and Their Paragenesis from the Sheared Granite of Gabal El Sela, South Eastern Desert, Egypt
Open Journal of Geology, 2020, 10, 703-725 https://www.scirp.org/journal/ojg ISSN Online: 2161-7589 ISSN Print: 2161-7570 Autunite-Group Minerals and Their Paragenesis from the Sheared Granite of Gabal El Sela, South Eastern Desert, Egypt Ehab K. Abu Zeid Nuclear Material Authority, Cairo, Egypt How to cite this paper: Zeid, E.K.A. (2020) Abstract Autunite-Group Minerals and Their Para- genesis from the Sheared Granite of Gabal G. El Sela is located in the Southern Eastern Desert of Egypt cropping as two El Sela, South Eastern Desert, Egypt. Open parts, occupied by monzogranites that were categorized as biotite granite, Journal of Geology, 10, 703-725. muscovite granite and two mica granites. The northern part is more signifi- https://doi.org/10.4236/ojg.2020.106031 cant according its high concentrations of uranium that controlled by compli- Received: April 27, 2020 cated structure regime; faulting, infrastructures and shearing are the most Accepted: June 27, 2020 common structural criteria of this part. The Egyptian Nuclear Materials Au- Published: June 30, 2020 thority (NMA) mined this part to produce the uranium ore. The previous mineralogical studies indicated that this granite was dominated by primary Copyright © 2020 by author(s) and Scientific Research Publishing Inc. uranium minerals (pitchblende and coffinite) and secondary minerals belong This work is licensed under the Creative to the autunite group (autunite, metautunite, phurcalite) in addition to ura- Commons Attribution-NonCommercial nophane. In the present work, petrographic and mineralogical studies are ap- International License (CC BY-NC 4.0). plied for the granites using the polarized and stereo microscopes and followed http://creativecommons.org/licenses/by-nc/4.0/ by electron microscope and XRD.