Binary Orbits As the Driver of Gamma-Ray Emission and Mass
Total Page:16
File Type:pdf, Size:1020Kb
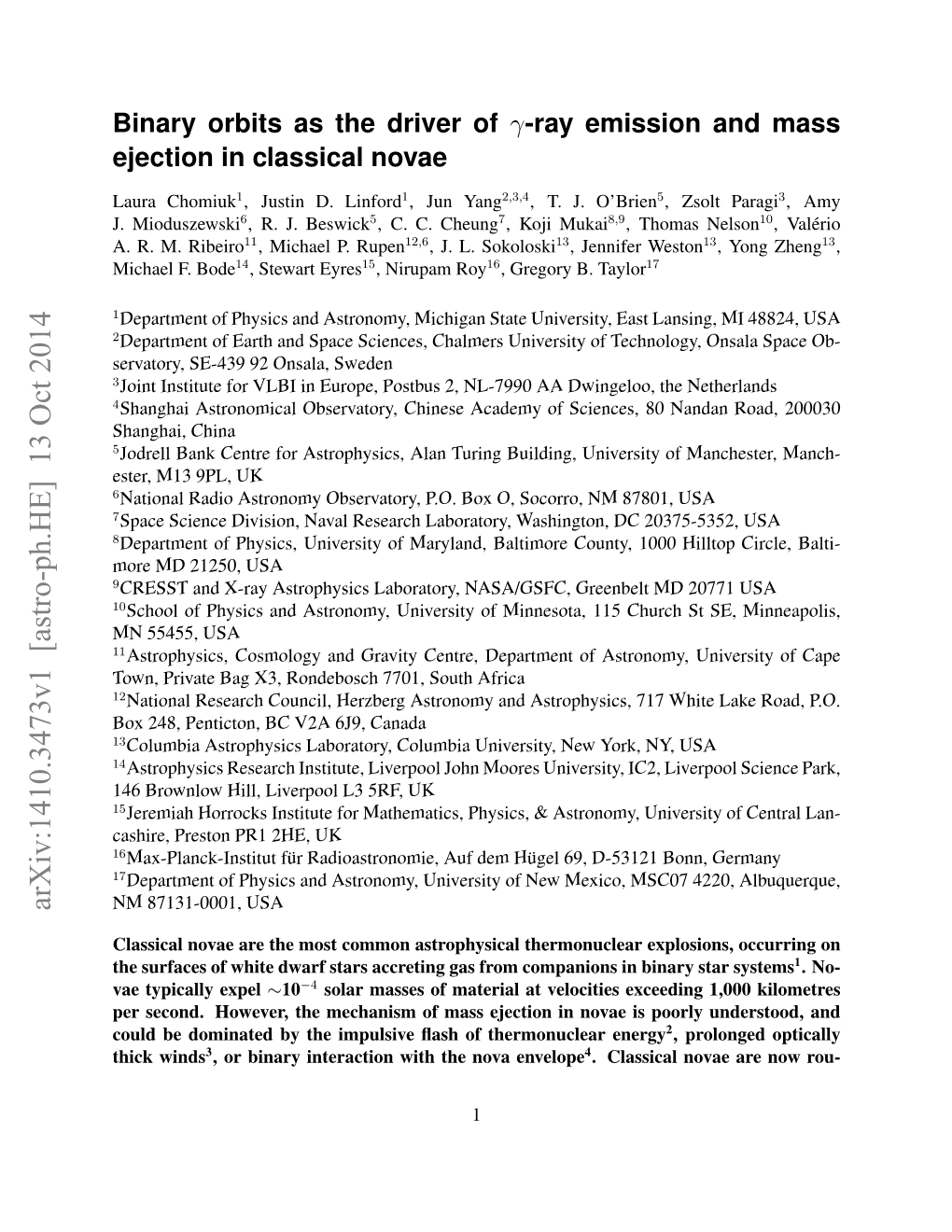
Load more
Recommended publications
-
Meeting Program
A A S MEETING PROGRAM 211TH MEETING OF THE AMERICAN ASTRONOMICAL SOCIETY WITH THE HIGH ENERGY ASTROPHYSICS DIVISION (HEAD) AND THE HISTORICAL ASTRONOMY DIVISION (HAD) 7-11 JANUARY 2008 AUSTIN, TX All scientific session will be held at the: Austin Convention Center COUNCIL .......................... 2 500 East Cesar Chavez St. Austin, TX 78701 EXHIBITS ........................... 4 FURTHER IN GRATITUDE INFORMATION ............... 6 AAS Paper Sorters SCHEDULE ....................... 7 Rachel Akeson, David Bartlett, Elizabeth Barton, SUNDAY ........................17 Joan Centrella, Jun Cui, Susana Deustua, Tapasi Ghosh, Jennifer Grier, Joe Hahn, Hugh Harris, MONDAY .......................21 Chryssa Kouveliotou, John Martin, Kevin Marvel, Kristen Menou, Brian Patten, Robert Quimby, Chris Springob, Joe Tenn, Dirk Terrell, Dave TUESDAY .......................25 Thompson, Liese van Zee, and Amy Winebarger WEDNESDAY ................77 We would like to thank the THURSDAY ................. 143 following sponsors: FRIDAY ......................... 203 Elsevier Northrop Grumman SATURDAY .................. 241 Lockheed Martin The TABASGO Foundation AUTHOR INDEX ........ 242 AAS COUNCIL J. Craig Wheeler Univ. of Texas President (6/2006-6/2008) John P. Huchra Harvard-Smithsonian, President-Elect CfA (6/2007-6/2008) Paul Vanden Bout NRAO Vice-President (6/2005-6/2008) Robert W. O’Connell Univ. of Virginia Vice-President (6/2006-6/2009) Lee W. Hartman Univ. of Michigan Vice-President (6/2007-6/2010) John Graham CIW Secretary (6/2004-6/2010) OFFICERS Hervey (Peter) STScI Treasurer Stockman (6/2005-6/2008) Timothy F. Slater Univ. of Arizona Education Officer (6/2006-6/2009) Mike A’Hearn Univ. of Maryland Pub. Board Chair (6/2005-6/2008) Kevin Marvel AAS Executive Officer (6/2006-Present) Gary J. Ferland Univ. of Kentucky (6/2007-6/2008) Suzanne Hawley Univ. -
FY1995 Q1 Oct-Dec NO
NATIONAL OPTICAL ASTRONOMY OBSERVATORIES NATIONAL OPTICAL ASTRONOMY OBSERVATORIES QUARTERLY REPORT OCTOBER - DECEMBER 1994 March 6, 1995 TABLE OF CONTENTS I. INTRODUCTION 1 II. SCIENTIFIC HIGHLIGHTS 1 A. Cerro Tololo Inter-American Observatory 1 1. CN and CH Abundance Variations in Globular Cluster Turnoff Stars and Implications for Stellar Evolution and Star Formation 1 2. Detection ofLens Candidates for the Double Quasar Q2345+007 2 3. The Lithium Abundance in M67 and Implications for Stellar Evolution and Cosmology 2 B. Kitt Peak National Observatory 3 1. Color Evolution of Galaxies 3 2. Kinematics ofBrightest Cluster Galaxies 4 3. Evolution ofthe Infrared Spectrum of an ONeMgNova 5 C. National Solar Observatory 6 1. High Temperature Coronal Regions Follow Solar Ring Current 6 2. Scattering ofP-Modes by Localized Inhomogeneities 7 3. Detection of Intrinsically Weak Solar Disk Magnetism 8 III. US GEMINI PROGRAM 9 IV. PERSONNEL AND BUDGET STATISTICS, NOAO 10 A. Visiting Scientists 10 B. Hired 10 C. Completed Employment 10 D. Changed Status 10 E. Gemini 8-m Telescopes Project 11 F. Chilean Economic Statistics 11 G. NSF Foreign Travel Fund 11 Appendices Appendix A: Telescope Usage Statistics Appendix B: Observational Programs Appendix C: Safety Report I. INTRODUCTION This document covers scientific highlights and personnel changes for the period 1 October - 31 December 1994. Highlights emphasize concluded projects rather than work in progress. The NOAO Newsletter Number 41 (March 1995) contains information on major projects, new instrumentation, and operations. The appendices to this report summarize telescope usage statistics and observational programs. H. SCIENTIFIC HIGHLIGHTS A. Cerro Tololo Inter-American Observatory 1. -
Binary Orbits As the Driver of Gamma
LETTER doi:10.1038/nature13773 Binary orbits as the driver of c-ray emission and mass ejection in classical novae Laura Chomiuk1, Justin D. Linford1, Jun Yang2,3,4, T. J. O’Brien5, Zsolt Paragi3, Amy J. Mioduszewski6, R. J. Beswick5, C. C. Cheung7, Koji Mukai8,9, Thomas Nelson10, Vale´rio A. R. M. Ribeiro11, Michael P. Rupen6,12, J. L. Sokoloski13, Jennifer Weston13, Yong Zheng13, Michael F. Bode14, Stewart Eyres15, Nirupam Roy16 & Gregory B. Taylor17 Classical novae are the most common astrophysical thermonuclear These observations span a frequency range of 1–6 GHz and show a flat a explosions, occurring on the surfaces of white dwarf stars accreting radio spectrum (a < 20.1, where Sn / n ; n is the observing frequency 1 gas from companions in binary star systems . Novae typically expel and Sn is the flux density at this frequency). This spectral index is much about 1024 solar masses of material atvelocitiesexceeding 1,000 kilo- more consistent with synchrotron radiation than the expected optic- metres per second. However, the mechanism of mass ejection in novae ally thick emission from warm nova ejecta13,14 (a < 2 is predicted and is poorly understood, and could be dominated by the impulsive flash observed in V959 Mon at later times; Fig. 1 and Extended Data Fig. 1). of thermonuclear energy2, prolonged optically thick winds3 or bin- Like gigaelectronvolt c-rays, synchrotron emission requires a popu- ary interaction with the nova envelope4. Classical novae are now lation of relativistic particles, and so we can use this radio emission as a routinely detected at gigaelectronvolt c-ray wavelengths5, suggest- tracer of c-ray production that lasts longer and enables much higher ing that relativistic particles are accelerated by strong shocks in the spatial resolution than do the c-rays themselves. -
Appendix II. Publications
Appendix II. Publications Gemini Staff Publications Papers in PeerReviewed Journals: Bauer, Amanda[4]. A young, dusty, compact radio source within a Lyα halo. Monthly Notices of the Royal Astronomical Society, 389:792-798. September, 2008. Trancho, G.[4]. The early expansion of cluster cores. Monthly Notices of the Royal Astronomical Society, 389:223-230. September, 2008. Stephens, A. W.[11]. Massive stars exploding in a He-rich circumstellar medium - III. SN 2006jc: infrared echoes from new and old dust in the progenitor CSM. Monthly Notices of the Royal Astronomical Society, 389:141-155. September, 2008. Serio, Andrew W.[1]. The variation of Io's auroral footprint brightness with the location of Io in the plasma torus. Icarus, 197:368-374. September, 2008. Radomski, James T.[5]. Understanding the 8 µm versus Paα Relationship on Subarcsecond Scales in Luminous Infrared Galaxies. The Astrophysical Journal, 685:211-224. September, 2008. Volk, K.[47]. Spitzer Survey of the Large Magellanic Cloud, Surveying the Agents of a Galaxy's Evolution (sage). IV. Dust Properties in the Interstellar Medium. The Astronomical Journal, 136:919-945. September, 2008. Díaz, R. J.[3]. Discovery of a [WO] central star in the planetary nebula Th 2-A. Astronomy and Astrophysics, 488:245-247. September, 2008. Schiavon, Ricardo P.[2]. Measuring Ages and Elemental Abundances from Unresolved Stellar Populations: Fe, Mg, C, N, and Ca. The Astrophysical Journal Supplement Series, 177:446- 464. August, 2008. Song, Inseok[3]. Gas and Dust Associated with the Strange, Isolated Star BP Piscium. The Astrophysical Journal, 683:1085-1103. August, 2008. Roth, Katherine C.[22]. -
Joint Meeting of the American Astronomical Society & The
American Association of Physics Teachers Joint Meeting of the American Astronomical Society & Joint Meeting of the American Astronomical Society & the 5-10 January 2007 / Seattle, Washington Final Program FIRST CLASS US POSTAGE PAID PERMIT NO 1725 WASHINGTON DC 2000 Florida Ave., NW Suite 400 Washington, DC 20009-1231 MEETING PROGRAM 2007 AAS/AAPT Joint Meeting 5-10 January 2007 Washington State Convention and Trade Center Seattle, WA IN GRATITUDE .....2 Th e 209th Meeting of the American Astronomical Society and the 2007 FOR FURTHER Winter Meeting of the American INFORMATION ..... 5 Association of Physics Teachers are being held jointly at Washington State PLEASE NOTE ....... 6 Convention and Trade Center, 5-10 January 2007, Seattle, Washington. EXHIBITS .............. 8 Th e AAS Historical Astronomy Divi- MEETING sion and the AAS High Energy Astro- REGISTRATION .. 11 physics Division are also meeting in LOCATION AND conjuction with the AAS/AAPT. LODGING ............ 12 Washington State Convention and FRIDAY ................ 44 Trade Center 7th and Pike Streets SATURDAY .......... 52 Seattle, WA AV EQUIPMENT . 58 SUNDAY ............... 67 AAS MONDAY ........... 144 2000 Florida Ave., NW, Suite 400, Washington, DC 20009-1231 TUESDAY ........... 241 202-328-2010, fax: 202-234-2560, [email protected], www.aas.org WEDNESDAY..... 321 AAPT AUTHOR One Physics Ellipse INDEX ................ 366 College Park, MD 20740-3845 301-209-3300, fax: 301-209-0845 [email protected], www.aapt.org Acknowledgements Acknowledgements IN GRATITUDE AAS Council Sponsors Craig Wheeler U. Texas President (6/2006-6/2008) Ball Aerospace Bob Kirshner CfA Past-President John Wiley and Sons, Inc. (6/2006-6/2007) Wallace Sargent Caltech Vice-President National Academies (6/2004-6/2007) Northrup Grumman Paul Vanden Bout NRAO Vice-President (6/2005-6/2008) PASCO Robert W. -
INES Access Guide No. 3 Classical Novae
INES Access Guide No. 3 Classical Novae Compiled by: Angelo Cassatella Consiglio Nazionale delle Ricerche IFSI (Roma) and Rosario Gonz´alez-Riestra XMM Science Operations Centre ESAC (Madrid) and Pierluigi Selvelli Consiglio Nazionale delle Ricerche IASF (Roma), INAF-OAT (Trieste) Scientific Coordinator for the INES Acess Guides Dr. Willem Wamsteker 1 2 FOREWORD The INES Access Guides The International Ultraviolet Explorer (IUE) Satellite (launched on 26 January 1978 and in or- bit until September 1996) Project was a collaboration between NASA, ESA and the PPARC. The IUE Spacecraft and instruments were operated in a Guest Observer mode to allow Ultraviolet Spec- trophotometry at two resolutions in the wavelength range from 115nm to 320nm: low resolution ∆λ/λ=300 (≈1000 km sec−1) and a high resolution mode ∆λ/λ=10000 (≈19 km sec−1). The IUE S/C, its scientific instruments as well as the data acquisition and reduction procedures, have been described in “Exploring the Universe with the IUE Satellite”, Part I, Part VI and Part VII (ASSL, volume 129, Eds. Y. Kondo, W. Wamsteker, M. Grewing, Kluwer Acad. Publ. Co.). A complete overview of the IUE Project is given in the conference proceedings of the last IUE Conference “Ultra- violet Astrophysics beyond the IUE Final Archive” (ESA SP-413, 1998, Eds. W. Wamsteker and R. Gonz´alezRiestra) and in “IUE Spacecraft Operations Final Report” (ESA SP-1215, 1997, A. P´erez Calpena and J. Pepoy). Additional information on the IUE Project and its data Archive INES can be found at URL http://sdc.laeff.esa.es. From the very beginning of the project, it was expected that the archival value of the data obtained with IUE would be very high. -
Annual Report / Rapport Annuel / Jahresbericht 1998
Annual Report / Rapport annuel / Jahresbericht 1998 EUROPEAN SOUTHERN OBSERVATORY COVER COUVERTURE UMSCHLAG Impressive images were obtained soon after Des images impressionnantes ont été reçues Beeindruckende Aufnahmen wurden ge- the multi-mode VLT Infrared Spectrometer peu de temps après l’installation de l’ins- macht kurz nachdem im November 1998 And Array Camera (ISAAC) instrument trument multi-mode ISAAC (« Infrared das Multimodus-Instrument ISAAC („Infra- was mounted at the first VLT 8.2-m Unit Spectrometer And Array Camera») au pre- red Spektrometer And Array Camera“) am mier télescope VLT de 8,2 mètres (UT1) en Telescope (UT1) in November 1998. This ersten der VLT-Teleskope (UT1) installiert colour composite image of the RCW38 star- novembre 1998. Cette image couleur com- worden war. Diese Farbaufnahme des forming complex combines Z (0.95 µm), H posite du complexe de formation stellaire (1.65 µm) and Ks (2.16 µm) exposures of a RCW38 combine des images prises en Z Sternentstehungsgebiets RCW38 ist zusam- few minutes each. Stars which have recent- (0,95 µm), H (1,65 µm) et Ks (2,16 µm), mengesetzt aus Einzelaufnahmen in Z (0,95 ly formed in clouds of gas and dust in this ayant un temps d’exposition de quelques µm), H (1,65 µm) und Ks (2,16 µm) von je region 5000 light-years away are still heav- minutes chacune. Les étoiles, récemment einigen Minuten Belichtungszeit. Die vor ily obscured and cannot be seen at optical formées dans les nuages de gaz et de pous- kurzem in den Gas- und Staubwolken dieser wavelengths but become visible at infrared sières dans cette région éloignée de 5000 5000 Lichtjahre entfernten Gegend ent- wavelengths where the obscuration is sub- années-lumière, sont extrêmement enfouies standenen Sterne sind immer noch stark stantially lower. -
Annual Report Rapport Annuel Jahresbericht 1997
Annual Report Rapport annuel Jahresbericht 1997 E U R O P E A N S O U T H E R N O B S E R V A T O R Y COVER COUVERTURE UMSCHLAG In December 1997, the first VLT 8.2-m mir- En décembre 1997, le premier miroir de Im Dezember 1997 erreichte der erste 8,2- ror arrived at the port of Antofagasta. The 8.20 m du VLT arriva au port d’Antofagasta. m-Spiegel für das VLT den Hafen von An- photograph shows the transport box with La photo montre la caisse de transport avec tofagasta. Diese Aufnahme zeigt, wie die the M1 mirror being unloaded from the ship le miroir en train d’être débarqué du bateau Transportkiste mit dem Spiegel vom Schiff M/S Tarpon Santiago. M/S Tarpon Santiago. M/S Tarpon Santiago entladen wird. Annual Report / Rapport annuel / Jahresbericht 1997 presented to the Council by the Director General présenté au Conseil par le Directeur général dem Rat vorgelegt vom Generaldirektor Prof. Dr. R. Giacconi E U R O P E A N S O U T H E R N O B S E R V A T O R Y Organisation Européenne pour des Recherches Astronomiques dans l’Hémisphère Austral Europäische Organisation für astronomische Forschung in der südlichen Hemisphäre Table Table Inhalts- of Contents des matières verzeichnis FOREWORD ................................. 5 PRÉFACE ...................................... 5 VORWORT ................................... 5 INTRODUCTION ......................... 7 INTRODUCTION ......................... 7 EINLEITUNG ............................... 7 RESEARCH HIGHLIGHTS ......... 9 POINTS CULMINANTS HÖHEPUNKTE Symposia and Workshops .............. 22 DE RECHERCHE ..................... 9 DER FORSCHUNG .................. 9 Conférences et colloques .............. -
ROBERT WILLIAMS Williams, RE 1965
Bibliography – ROBERT WILLIAMS Williams, R.E. 1965, "The Size of the Solar HII Region," Ap. J., 142, 314. Williams, R.E. 1967, "The Ionization and Thermal Equilibrium of a Gas Excited by Ultraviolet Synchrotron Radiation," Ap. J., 147, 556. Williams, R.E. 1968, "The Ionization of Planetary Nebulae," in IAU Symp. No. 34: Planetary Nebulae, eds. D.E. Osterbrock and C.R. O'Dell (Dordrecht: Reidel), p. 190. Williams, R.E., and Weymann, R.J. 1968, "Calculated Line Intensities for Models of Seyfert Galaxy Nuclei," Astron. J., 73, 895. Williams, R.E. 1968, "The Nuclei of Seyfert Galaxies," A.S.P. Leaflet No. 473. Henry, R.J.W., and Williams, R.E. 1968, "Collision Strengths and Photoionization Cross Sections for Nitrogen, Oxygen, and Neon," Pub. A.S.P., 80, 669. Weymann, R.J., and Williams, R.E. 1969, "The Bowen Fluorescence Mechanism in Planetary Nebulae and the Nuclei of Seyfert Galaxies," Ap. J., 157, 1201. Williams, R.E. 1970, "[O I] 6300 Emission in Planetary Nebulae," Ap. J., 159, 829. Berry, H.G., Bickel, W., Martinson, I., Weymann, R.J., and Williams, R.E. 1970, "Total Transition Probabilities for the Bowen Levels of O III," Ap. Lett., 5, 81. Williams, R.E. 1970, "Absorption Lines in Quasi-Stellar Objects with zabs>zem," Nature, 228, 269. Capriotti, E.R., Cromwell, R.H., and Williams, R.E. 1971, "Observed Small-Scale Structure in Planetary Nebulae," Ap. Lett., 7, 241. Williams, R.E. 1971, "The Helium Abundance in Quasi-Stellar Objects," Ap. J. Lett., 167, L27. Williams, R.E. 1972, "The Effects of Radiation Pressure from Resonance Scattering in a Quasar Cloud," Ap. -
Assoc. Prof. Hasan Hüseyin ESENOĞLU
Assoc. Prof. Hasan Hüseyin ESENOĞLU MPeorbsiloen Pahlo Innef:o +r9m0a 5t4i5o n341 6086 Office Phone: +90 2412 242470 20307050 Extension: 10291 EFmaxa iPl:h oesneen:o +gl9u0@ 2is1t2an 4b4u0l. e0d3u7.0tr AWdedbr:e hstst:p :İ/s/tawnwbuwl. iÜstnainvebrusli.etedsui .Gtrö/zfelenm/aesvtir 3o4n1o1m9y, /Fhaitnihfo, B.pehypa?ziınt,f oİs=ta8n&bsuelt=1 BHaiosagnr Ha.p Ehseynoğlu was born on 28 February 1963 in Eskişehir. finished Eskişehir Technical High School Machine FDaecpualrtytm. Heen tg. rIand 1u9a8te2d, hine 1en9t8e6r e(db athchee Dloerp daretgmreen)t aonf dA sintr 1o9n9o0m (ym aansdt eSrp daceeg rSeceie)n. Icne s1 9at9 I6s thaen bcouml Upnleivtedrs hitiys Sdcoicetnocreate at athttee nsadmede tuhnei vAesrisaigtyo. Ast rao Fpehllyoswicsahl iOpb osfe trhvea tMorinyi sotfr Py aodfo Fvoar Ueinginv eArfsfiatiyr sin o Ift athlye fIotarl itawno M yienairsstr bye ofof rFeo raenidg na fAteffra hiriss, he Idnovcetsotrigaateto. 3r ayte aTrÜsB SİaTuAdKi NAraatiboinaa (lR Oiybaste)r vKaintogr Sya (u2d0 U11n-iv1e7r)s. iTtyh eY aarrdti.cDleosç .pDurb. (li2s0h0ed4 -i0n7 t)h aen SdC I5 i nyedaerxse sa sh Pavrien bciepeanl published. aTshseisrtea anrt ep irnovfestsiogra taiot nIsst aonnb Nuol Uvaneiv, ecarstaitcyl,y Fsmaciuc lvtya roifa bScleie sntcaer.s and meteorites. He has been currently working as an EDodcutocraatteio, İnst aInnbfuol rÜmniavetriosintesi, Institute of Graduate Studies In Sciences, Astronomi Ve Uzay Bilimleri Bölümü Yıldız PAostsrtogrfiazdiğui aPtreo, İgsrtaamnbı,u Tl uÜrnkievye r1s9it9e0s i-, I1n9s9ti6tute of Graduate Studies In Sciences, -
THE NEON ABUNDANCE in the EJECTA of QU VULPECULAE from LATE-EPOCH INFRARED SPECTRA Robert D
The Astrophysical Journal, 672:1167Y1173, 2008 January 10 # 2008. The American Astronomical Society. All rights reserved. Printed in U.S.A. THE NEON ABUNDANCE IN THE EJECTA OF QU VULPECULAE FROM LATE-EPOCH INFRARED SPECTRA Robert D. Gehrz,1 Charles E. Woodward,1 L. Andrew Helton,1 Elisha F. Polomski,1 Thomas L. Hayward,2 James R. Houck,3 A. Evans,4 Joachim Krautter,5 Steven N. Shore,6 Sumner Starrfield,7 James Truran,8 G. J. Schwarz,9 and R. Mark Wagner10 Received 2006 September 1; accepted 2007 September 14 ABSTRACT We present ground-based SpectroCam-10 mid-infrared, MMToptical, and Spitzer Space Telescope IRS mid-infrared spectra taken 7.62, 18.75, and 19.38 yr, respectively, after the outburst of the old classical nova QU Vulpeculae (Nova Vul 1984#2). The spectra of the ejecta are dominated by forbidden line emission from neon and oxygen. Our analysis shows that neon was, at the first and last epochs respectively, more than 76 and 168 times overabundant by number with respect to hydrogen compared to the solar value. These high lower limits to the neon abundance confirm that QU Vul involved a thermonuclear runaway on an ONeMg white dwarf, and approach the yields predicted by models of the nucleosynthesis in such events. Subject headinggs: infrared: stars — novae, cataclysmic variables — stars: individual (QU Vul, Nova Vul 1984 #2) 1. INTRODUCTION many important IR lines, the absolute numbers of atoms involved in these transitions can be calculated with reasonable certainty Gehrz et al. (1985) discovered a very strong [Ne ii]12.8 m given a known distance. -
Arxiv:2004.06540V2 [Astro-Ph.SR] 30 Apr 2020 2
The Astronomy and Astrophysics Review manuscript No. (will be inserted by the editor) Observations of galactic and extragalactic novae Massimo Della Valle and Luca Izzo Received: date / Accepted: date Abstract The recent GAIA DR2 measurements of distances to galactic novae have allowed to re-analyse some properties of nova populations in the Milky Way and in external galaxies on new and more solid empirical bases. In some cases we have been able to confirm results previously obtained, such as the concept of nova populations into two classes of objects, that is disk and bulge novae and their link with the Tololo spectroscopic classification in Fe II and He/N novae. The recent and robust estimates of nova rates in the Magellanic Clouds galaxies provided by the OGLE team have confirmed the dependence of the normalized nova rate (i.e., the nova rate per unit of luminosity of the host galaxy) with the colors and/or class of luminosity of the parent galaxies. The nova rates in the Milky Way and in external galaxies have been collected from literature and critically discussed. They are the necessary ingredient to asses the contribution of novae to the nucleosynthesis of the respective host galaxies, particularly to explain the origin of the overabundance of lithium observed in young stellar populations. A direct comparison between distances obtained via GAIA DR2 and Maximum Magnitude vs. Rate of Decline (MMRD) relation- ship points out that the MMRD can provide distances with an uncertainty better than 30%. Multiwavelength observations of novae along the whole elec- tromagnetic spectrum, from radio to gamma-rays, have revealed that novae undergo a complex evolution characterized by several emission phases and a non-spherical geometry for the nova ejecta.