Activation of Parkin Ching
Total Page:16
File Type:pdf, Size:1020Kb
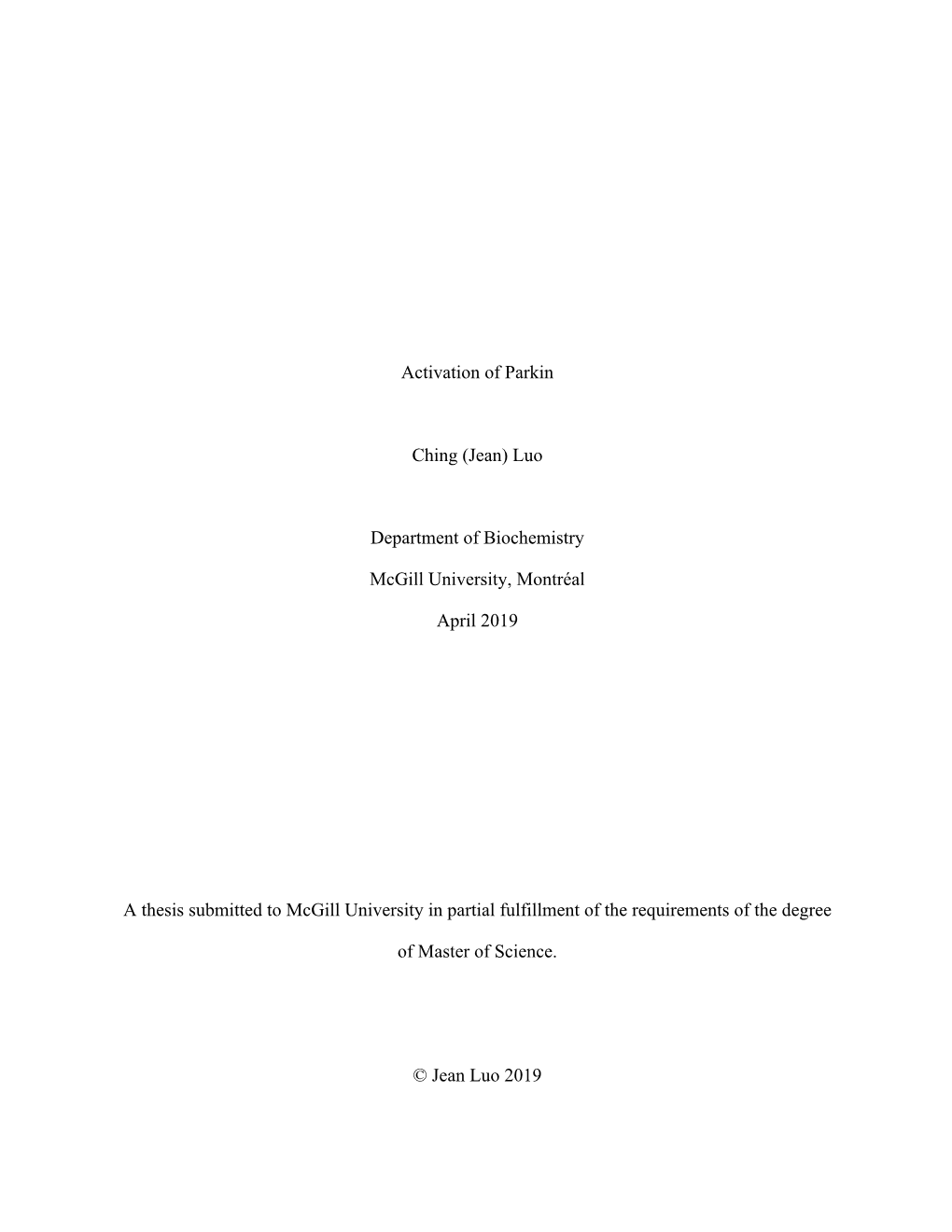
Load more
Recommended publications
-
PICK1-Deficient Mice Exhibit Impaired Response to Cocaine and Dysregulated Dopamine Homeostasis
PICK1-Deficient Mice Exhibit Impaired Response to Cocaine and Dysregulated Dopamine Homeostasis Jensen, Kathrine Louise; Sørensen, Gunnar; Dencker, Ditte; Owens, William Anthony; Rahbek-Clemmensen, Troels; Brett Lever, Michael; Runegaard, Annika H; Riis Christensen, Nikolaj; Weikop, Pia; Wörtwein, Gitta; Fink-Jensen, Anders; Madsen, Kenneth L; Daws, Lynette; Gether, Ulrik; Rickhag, Mattias Published in: eNeuro DOI: 10.1523/ENEURO.0422-17.2018 Publication date: 2018 Document version Publisher's PDF, also known as Version of record Document license: CC BY Citation for published version (APA): Jensen, K. L., Sørensen, G., Dencker, D., Owens, W. A., Rahbek-Clemmensen, T., Brett Lever, M., Runegaard, A. H., Riis Christensen, N., Weikop, P., Wörtwein, G., Fink-Jensen, A., Madsen, K. L., Daws, L., Gether, U., & Rickhag, M. (2018). PICK1-Deficient Mice Exhibit Impaired Response to Cocaine and Dysregulated Dopamine Homeostasis. eNeuro, 5(3). https://doi.org/10.1523/ENEURO.0422-17.2018 Download date: 25. sep.. 2021 New Research Disorders of the Nervous System PICK1-Deficient Mice Exhibit Impaired Response to Cocaine and Dysregulated Dopamine Homeostasis ء ء Kathrine Louise Jensen,1, Gunnar Sørensen,1,2, Ditte Dencker,2 William Anthony Owens,3 Troels Rahbek-Clemmensen,1 Michael Brett Lever,1 Annika H. Runegaard,1 Nikolaj Riis Christensen,1 Pia Weikop,2 Gitta Wörtwein,2 Anders Fink-Jensen,2 Kenneth L. Madsen,1 Lynette Daws,3 Ulrik Gether,1 and Mattias Rickhag1 DOI:http://dx.doi.org/10.1523/ENEURO.0422-17.2018 1Molecular Neuropharmacology and Genetics -
AMPA Receptor Dysregulation and Therapeutic Interventions in a Mouse Model of CDKL5 Deficiency Disorder
4814 • The Journal of Neuroscience, June 12, 2019 • 39(24):4814–4828 Neurobiology of Disease AMPA Receptor Dysregulation and Therapeutic Interventions in a Mouse Model of CDKL5 Deficiency Disorder Madhumita Yennawar,1 XRachel S. White,2 and XFrances E. Jensen2 1Department of Systems Pharmacology and Translational Therapeutics, and 2Department of Neurology, University of Pennsylvania Perelman School of Medicine, Philadelphia, Pennsylvania 19104 Pathogenic mutations in cyclin-dependent kinase-like 5 (CDKL5) result in CDKL5 deficiency disorder (CDD), a rare disease marked by early-life seizures, autistic behaviors, and intellectual disability. Although mouse models of CDD exhibit dendritic instability and alter- ations in synaptic scaffolding proteins, studies of glutamate receptor levels and function are limited. Here we used a novel mouse model of CDD, the Cdkl5R59X knock-in mouse (R59X), to investigate changes in synaptic glutamate receptor subunits and functional conse- quences. Male mice were used for all experiments to avoid the confounding effects of X-inactivation that would be present in female heterozygous mice. We showed that adult male R59X mice recapitulated the behavioral outcomes observed in other mouse models of CDD, including social deficits and memory and learning impairments, and exhibited decreased latency to seizure upon pentylenetetrazol administration. Furthermore, we observed a specific increase in GluA2-lacking ␣-amino-3-hydroxy-5-methyl-4-isoxazolepropionic acid)-type glutamate receptors (AMPARs) in the adult R59X hippocampus, which is accompanied electrophysiologically by increased rectification ratio of AMPAR EPSCs and elevated early-phase long term potentiation (LTP). Finally, we showed that acute treatment with the GluA2-lacking AMPAR blocker IEM-1460 decreased AMPAR currents, and rescued social deficits, working memory impairments, and seizure behavior latency in R59X mice. -
PICK1 Is Implicated in Organelle Motility in an Arp2/3 Complex–Independent Manner
M BoC | ARTICLE PICK1 is implicated in organelle motility in an Arp2/3 complex–independent manner Yadaiah Madasua, Changsong Yangb, Malgorzata Boczkowskaa, Kelley A. Bethoneya, Adam Zwolaka, Grzegorz Rebowskia, Tatyana Svitkinab, and Roberto Domingueza aDepartment of Physiology, Perelman School of Medicine, and bDepartment of Biology, University of Pennsylvania, Philadelphia, PA 19104 ABSTRACT PICK1 is a modular scaffold implicated in synaptic receptor trafficking. It features Monitoring Editor a PDZ domain, a BAR domain, and an acidic C-terminal tail (ACT). Analysis by small- angle x- Thomas D. Pollard ray scattering suggests a structural model that places the receptor-binding site of the PDZ Yale University domain and membrane-binding surfaces of the BAR and PDZ domains adjacent to each other Received: Oct 14, 2014 on the concave side of the banana-shaped PICK1 dimer. In the model, the ACT of one subunit Revised: Dec 23, 2014 of the dimer interacts with the PDZ and BAR domains of the other subunit, possibly account- Accepted: Jan 26, 2015 ing for autoinhibition. Consistently, full-length PICK1 shows diffuse cytoplasmic localization, but it clusters on vesicle-like structures that colocalize with the trans-Golgi network marker TGN38 upon deletion of either the ACT or PDZ domain. This localization is driven by the BAR domain. Live-cell imaging further reveals that PICK1-associated vesicles undergo fast, nondi- rectional motility in an F-actin–dependent manner, but deleting the ACT dramatically reduces vesicle speed. Thus the ACT links PICK1-associated vesicles to a motility factor, likely myosin, but, contrary to previous reports, PICK1 neither binds nor inhibits Arp2/3 complex. -
Human Induced Pluripotent Stem Cell–Derived Podocytes Mature Into Vascularized Glomeruli Upon Experimental Transplantation
BASIC RESEARCH www.jasn.org Human Induced Pluripotent Stem Cell–Derived Podocytes Mature into Vascularized Glomeruli upon Experimental Transplantation † Sazia Sharmin,* Atsuhiro Taguchi,* Yusuke Kaku,* Yasuhiro Yoshimura,* Tomoko Ohmori,* ‡ † ‡ Tetsushi Sakuma, Masashi Mukoyama, Takashi Yamamoto, Hidetake Kurihara,§ and | Ryuichi Nishinakamura* *Department of Kidney Development, Institute of Molecular Embryology and Genetics, and †Department of Nephrology, Faculty of Life Sciences, Kumamoto University, Kumamoto, Japan; ‡Department of Mathematical and Life Sciences, Graduate School of Science, Hiroshima University, Hiroshima, Japan; §Division of Anatomy, Juntendo University School of Medicine, Tokyo, Japan; and |Japan Science and Technology Agency, CREST, Kumamoto, Japan ABSTRACT Glomerular podocytes express proteins, such as nephrin, that constitute the slit diaphragm, thereby contributing to the filtration process in the kidney. Glomerular development has been analyzed mainly in mice, whereas analysis of human kidney development has been minimal because of limited access to embryonic kidneys. We previously reported the induction of three-dimensional primordial glomeruli from human induced pluripotent stem (iPS) cells. Here, using transcription activator–like effector nuclease-mediated homologous recombination, we generated human iPS cell lines that express green fluorescent protein (GFP) in the NPHS1 locus, which encodes nephrin, and we show that GFP expression facilitated accurate visualization of nephrin-positive podocyte formation in -
The Glutamate Receptor Ion Channels
0031-6997/99/5101-0007$03.00/0 PHARMACOLOGICAL REVIEWS Vol. 51, No. 1 Copyright © 1999 by The American Society for Pharmacology and Experimental Therapeutics Printed in U.S.A. The Glutamate Receptor Ion Channels RAYMOND DINGLEDINE,1 KARIN BORGES, DEREK BOWIE, AND STEPHEN F. TRAYNELIS Department of Pharmacology, Emory University School of Medicine, Atlanta, Georgia This paper is available online at http://www.pharmrev.org I. Introduction ............................................................................. 8 II. Gene families ............................................................................ 9 III. Receptor structure ...................................................................... 10 A. Transmembrane topology ............................................................. 10 B. Subunit stoichiometry ................................................................ 10 C. Ligand-binding sites located in a hinged clamshell-like gorge............................. 13 IV. RNA modifications that promote molecular diversity ....................................... 15 A. Alternative splicing .................................................................. 15 B. Editing of AMPA and kainate receptors ................................................ 17 V. Post-translational modifications .......................................................... 18 A. Phosphorylation of AMPA and kainate receptors ........................................ 18 B. Serine/threonine phosphorylation of NMDA receptors .................................. -
Alternative Splicing of AMPA Subunits in Prefrontal Cortical Fields Of
View metadata, citation and similar papers at core.ac.uk brought to you by CORE ORIGINAL RESEARCH ARTICLE published: 03 Januaryprovided 2012 by Frontiers - Publisher Connector PSYCHIATRY doi: 10.3389/fpsyt.2011.00072 Alternative splicing of AMPA subunits in prefrontal cortical fields of cynomolgus monkeys following chronic ethanol self-administration Glen Acosta1, David P.Freidman1, Kathleen A. Grant 2 and Scott E. Hemby 1,3* 1 Department of Physiology and Pharmacology, Wake Forest University, Winston-Salem, NC, USA 2 Oregon National Primate Research Center, Oregon Health and Sciences University, Beaverton, OR, USA 3 Department of Psychiatry and Behavioral Sciences, Wake Forest University, Winston-Salem, NC, USA Edited by: Functional impairment of the orbital and medial prefrontal cortex underlies deficits in exec- Lara Ray, University of California, USA utive control that characterize addictive disorders, including alcohol addiction. Previous Reviewed by: studies indicate that alcohol alters glutamate neurotransmission and one substrate of these Raj Sevak, University of California, USA effects may be through the reconfiguration of the subunits constituting ionotropic gluta- Shaolin Wang, University of Virginia, mate receptor (iGluR) complexes. Glutamatergic transmission is integral to cortico-cortical USA and cortico-subcortical communication and alcohol-induced changes in the abundance of *Correspondence: the receptor subunits and/or their splice variants may result in critical functional impair- Scott E. Hemby, Medical Center ments of prefrontal cortex in alcohol dependence. To this end, the effects of chronic Boulevard, Wake Forest University School of Medicine, Winston-Salem, ethanol self-administration on glutamate receptor ionotropic AMPA (GRIA) subunit vari- NC 27157, USA. ant and kainate (GRIK) subunit mRNA expression were studied in the orbitofrontal cortex e-mail: [email protected] (OFC), dorsolateral prefrontal cortex (DLPFC), and anterior cingulate cortex (ACC) of male cynomolgus monkeys. -
UNIVERSITY of CALIFORNIA, SAN DIEGO the Role of PICK1 And
UNIVERSITY OF CALIFORNIA, SAN DIEGO The Role of PICK1 and PKC alpha in Alzheimer’s Disease A dissertation submitted in partial satisfaction of the requirements for the degree Doctor of Philosophy in Neurosciences by Stephanie Isabel Alfonso Committee in charge: Professor Roberto Malinow, Chair Professor Andrea Chiba Professor Todd Coleman Professor Fred Gage Professor Gentry Patrick 2016 The Dissertation of Stephanie Isabel Alfonso is approved, and it is acceptable in quality and form for publication on microfilm and electronically: Chair University of California, San Diego 2016 iii DEDICATION I would like to dedicate this thesis to my son and inspiration, Alex Alfonso. Thank you for changing my life and giving me a purpose to live. It is your love and your smile that keep me going everyday and I could never imagine a life without you. This will be the beginning of a major turning point in our lives and I’m glad you’ll be there to share it with me. Tambien le dedico esta tésis a mis padres y mi hermano que siempre han estado alli para ayudarme con todo. Siempre han sido un gran apoyo y nunca les podre pagar lo que han hecho por mi. Gracias por todo el amor que me han dado a mi y a mi hijo. Los quiero mas a que a nadie en el mundo, Steph. iv TABLE OF CONTENTS Signature Page…………………………………………………………………… iii Dedication………………………………………………………………………... iv Table of Contents……………………………………………………………….... v List of Figures.…………………............................................................................ vi Acknowledgements………………………………………………………………. vii Vita………………………………………..…………………………………..….. viii Abstract of the Dissertation ……………………………………………………… ix Introduction………………………………………………………………………. 1 Chapter I- Synapto-depressive effects of Aβ require PICK1 …………………......3 Chapter II- PKCα’s role in Alzheimer’s Disease …………..……………………..20 Appendix…………………………………………………………………………. -
PICK1-Deficient Mice Exhibit Impaired Response to Cocaine and Dysregulated Dopamine Homeostasis
New Research Disorders of the Nervous System PICK1-Deficient Mice Exhibit Impaired Response to Cocaine and Dysregulated Dopamine Homeostasis ء ء Kathrine Louise Jensen,1, Gunnar Sørensen,1,2, Ditte Dencker,2 William Anthony Owens,3 Troels Rahbek-Clemmensen,1 Michael Brett Lever,1 Annika H. Runegaard,1 Nikolaj Riis Christensen,1 Pia Weikop,2 Gitta Wörtwein,2 Anders Fink-Jensen,2 Kenneth L. Madsen,1 Lynette Daws,3 Ulrik Gether,1 and Mattias Rickhag1 DOI:http://dx.doi.org/10.1523/ENEURO.0422-17.2018 1Molecular Neuropharmacology and Genetics Laboratory, Department of Neuroscience, Faculty of Health and Medical Sciences, University of Copenhagen, Copenhagen DK-2200, Denmark, 2Laboratory of Neuropsychiatry, Psychiatric Center Copenhagen, Faculty of Health and Medical Sciences, University of Copenhagen, Copenhagen DK-2200, Denmark, and 3Department of Cellular and Integrative Physiology, University of Texas Health Science Center at San Antonio, TX 78229 Abstract Protein interacting with C-kinase 1 (PICK1) is a widely expressed scaffold protein known to interact via its PSD-95/discs-large/ZO-1 (PDZ)-domain with several membrane proteins including the dopamine (DA) transporter (DAT), the primary target for cocaine’s reinforcing actions. Here, we establish the importance of PICK1 for behavioral effects observed after both acute and repeated administration of cocaine. In PICK1 knock-out (KO) mice, the acute locomotor response to a single injection of cocaine was markedly attenuated. Moreover, in support of a role for PICK1 in neuroadaptive changes induced by cocaine, we observed diminished cocaine intake in a self-administration paradigm. Reduced behavioral effects of cocaine were not associated with decreased striatal DAT distribution and most likely not caused by the ϳ30% reduction in synaptosomal DA uptake observed in PICK1 KO mice. -
Deletion of Glutamate Receptor Trafficking Proteins in the Medial Prefrontal Cortex and Their Sex-Specific Effects on Cocaine Addiction
DELETION OF GLUTAMATE RECEPTOR TRAFFICKING PROTEINS IN THE MEDIAL PREFRONTAL CORTEX AND THEIR SEX-SPECIFIC EFFECTS ON COCAINE ADDICTION A Dissertation Submitted to the Temple University Graduate Board In Partial Fulfillment of the Requirements for the Degree DOCTOR OF PHILOSOPHY by Megan Marie Wickens May 2020 Examining Committee Members: Dr. Lisa Briand, Advisory Chair, Temple University Psychology Department Dr. Debra Bangasser, Temple University Psychology Department Dr. Vinay Parikh, Temple University Psychology Department Dr. Mathieu Wimmer, Temple University Psychology Department Dr. Vishnu Murty, Temple University Psychology Department Dr. Scott Rawls, Temple University Pharmacology Department ABSTRACT Dysregulation of glutamatergic signaling mechanisms is a component of many psychiatric diseases. A number of these diseases exhibit a bias toward one sex, yet the ways in which glutamate is affected by or modulates this bias is poorly understood. In cocaine addiction, women progress from initial use of the drug to substance use disorder faster than men, and have more difficulty remaining abstinent. The same is true in female rodents. We used a mouse model of cocaine self-administration to study the role of glutamate receptor trafficking proteins in cocaine addiction-like behavior in males and females. In the first set of experiments, mice received a conditional knockout of glutamate receptor interacting protein 1 (GRIP1) in the medial prefrontal cortex (mPFC). This led to an increase in motivation for cocaine as well as enhanced likelihood of relapse behavior, as measured by a progressive ratio schedule and cue-induced reinstatement, respectively. No sex differences were seen after prefrontal deletion of GRIP1. The next set of experiments used the same behavioral paradigm, but mice received a conditional knockout of protein interacting with C kinase 1 (PICK1) in the mPFC. -
Ampa Receptor Dysregulation and Therapeutic Interventions in a Mouse Model of Cdkl5 Deficiency Disorder
University of Pennsylvania ScholarlyCommons Publicly Accessible Penn Dissertations 2018 Ampa Receptor Dysregulation And Therapeutic Interventions In A Mouse Model Of Cdkl5 Deficiency Disorder Madhumita Yennawar University of Pennsylvania, [email protected] Follow this and additional works at: https://repository.upenn.edu/edissertations Part of the Neuroscience and Neurobiology Commons Recommended Citation Yennawar, Madhumita, "Ampa Receptor Dysregulation And Therapeutic Interventions In A Mouse Model Of Cdkl5 Deficiency Disorder" (2018). Publicly Accessible Penn Dissertations. 3209. https://repository.upenn.edu/edissertations/3209 This paper is posted at ScholarlyCommons. https://repository.upenn.edu/edissertations/3209 For more information, please contact [email protected]. Ampa Receptor Dysregulation And Therapeutic Interventions In A Mouse Model Of Cdkl5 Deficiency Disorder Abstract CDKL5 Deficiency Disorder (CDD) is a rare disease that presents as a set of neurological deficits including early-life epilepsy, intellectual disability, and autistic-like behaviors. It results from pathogenic mutations in the gene for cyclin-dependent kinase-like 5 (CDKL5), a protein that is highly expressed in brain. There is no cure for CDD and seizures in this disorder are typically resistant to traditional anti-epileptic drugs, although some patients respond well to cannabidiol. However, underlying mechanisms of what causes hyperexcitability and neurological deficits in CDD is poorly understood. We investigated the novel Cdkl5R59X mouse (R59X), and observed that mutant mice have social interaction and memory deficits, and decreased latency to seizure after administration of pentylenetetrazol. Given the observed behavioral alterations and hyperexcitability in R59X mice, we hypothesized that mutant mice would exhibit underlying molecular and functional alterations in proteins involved in regulating the E:I balance. -
Trafficking of Kainate Receptors
Membranes 2014, 4, 565-595; doi:10.3390/membranes4030565 OPEN ACCESS membranes ISSN 2077-0375 www.mdpi.com/journal/membranes Review Trafficking of Kainate Receptors Steffen Pahl 1;2;3, Daniel Tapken 1, Simon C. Haering 1;3;4 and Michael Hollmann 1;2;* 1 Department of Biochemistry I, Ruhr University Bochum, Universitätsstr. 150, 44780 Bochum, Germany; E-Mails: [email protected] (S.P.); [email protected] (D.T.); [email protected] (S.C.H.) 2 International Graduate School of Neuroscience, Ruhr University Bochum, Universitätsstr. 150, 44780 Bochum, Germany 3 Ruhr University Research School, Ruhr University Bochum, Universitätsstr. 150, 44780 Bochum, Germany 4 Graduate School of Chemistry and Biochemistry, Ruhr University Bochum, Universitätsstr. 150, 44780 Bochum, Germany * Author to whom correspondence should be addressed; E-Mail: [email protected]; Tel.: +49-234-32-28225; Fax: +49-234-32-14244. Received: 6 July 2014; in revised form: 4 August 2014 / Accepted: 12 August 2014 / Published: 20 August 2014 Abstract: Ionotropic glutamate receptors (iGluRs) mediate the vast majority of excitatory neurotransmission in the central nervous system of vertebrates. In the protein family of iGluRs, kainate receptors (KARs) comprise the probably least well understood receptor class. Although KARs act as key players in the regulation of synaptic network activity, many properties and functions of these proteins remain elusive until now. Especially the precise pre-, extra-, and postsynaptic localization of KARs plays a critical role for neuronal function, as an unbalanced localization of KARs would ultimately lead to dysregulated neuronal excitability. Recently, important advances in the understanding of the regulation of surface expression, function, and agonist-dependent endocytosis of KARs have been achieved. -
SUSD4 Controls Activity-Dependent Degradation of AMPA Receptor GLUA2 and Synaptic Plasticity
bioRxiv preprint doi: https://doi.org/10.1101/859587; this version posted November 29, 2019. The copyright holder for this preprint (which was not certified by peer review) is the author/funder. All rights reserved. No reuse allowed without permission. SUSD4 Controls Activity-Dependent Degradation of AMPA Receptor GLUA2 and Synaptic Plasticity I. González-Calvo1,2,†, K. Iyer1,†, M. Carquin1, A. Khayachi1, F.A. Giuliani2, J. Vincent3, M. Séveno4, S.M. Sigoillot1, M. Veleanu1, S. Tahraoui1, M. Albert1, O. Vigy5, Y. Nadjar6, A. Dumoulin6, A. Triller6, J.-L. Bessereau7, L. Rondi-Reig3, P. Isope2, F. Selimi1* Affiliations: 1 Center for Interdisciplinary Research in Biology (CIRB), Collège de France, CNRS, INSERM, PSL Research University, Paris, France. 2 Institut de Neurosciences Cellulaires et Intégratives (INCI), CNRS, Université de Strasbourg, , Strasbourg, France. 3 Institut Biology Paris Seine (IBPS), Neuroscience Paris Seine (NPS), CeZaMe, CNRS, Sorbonne University, INSERM, Paris, France. 4 BioCampus Montpellier, CNRS, INSERM, Université de Montpellier, Montpellier, France. 5 Institut de Génomique Fonctionnelle, CNRS, INSERM, Université de Montpellier, Montpellier, France. 6 École Normale Supérieure, Institut de Biologie de l'ENS, INSERM, CNRS, PSL Research University, Paris, France. 7 Université de Lyon, Université Claude Bernard Lyon 1, CNRS UMR 5310, INSERM U 1217, Institut Neuromyogène, 69008, Lyon, France. * Correspondence to: [email protected] † Equal contribution. Summary At excitatory synapses, the choice between recycling or degradation of glutamate AMPA receptors controls the direction of synaptic plasticity. In this context, how the degradation machinery is targeted to specific synaptic substrates in an activity-dependent manner is not understood. Here we show that SUSD4, a complement-related transmembrane protein, is a tether for HECT ubiquitin ligases of the NEDD4 subfamily, which promote the degradation of a large number of cellular substrates.