Osu1143214139.Pdf (10.83
Total Page:16
File Type:pdf, Size:1020Kb
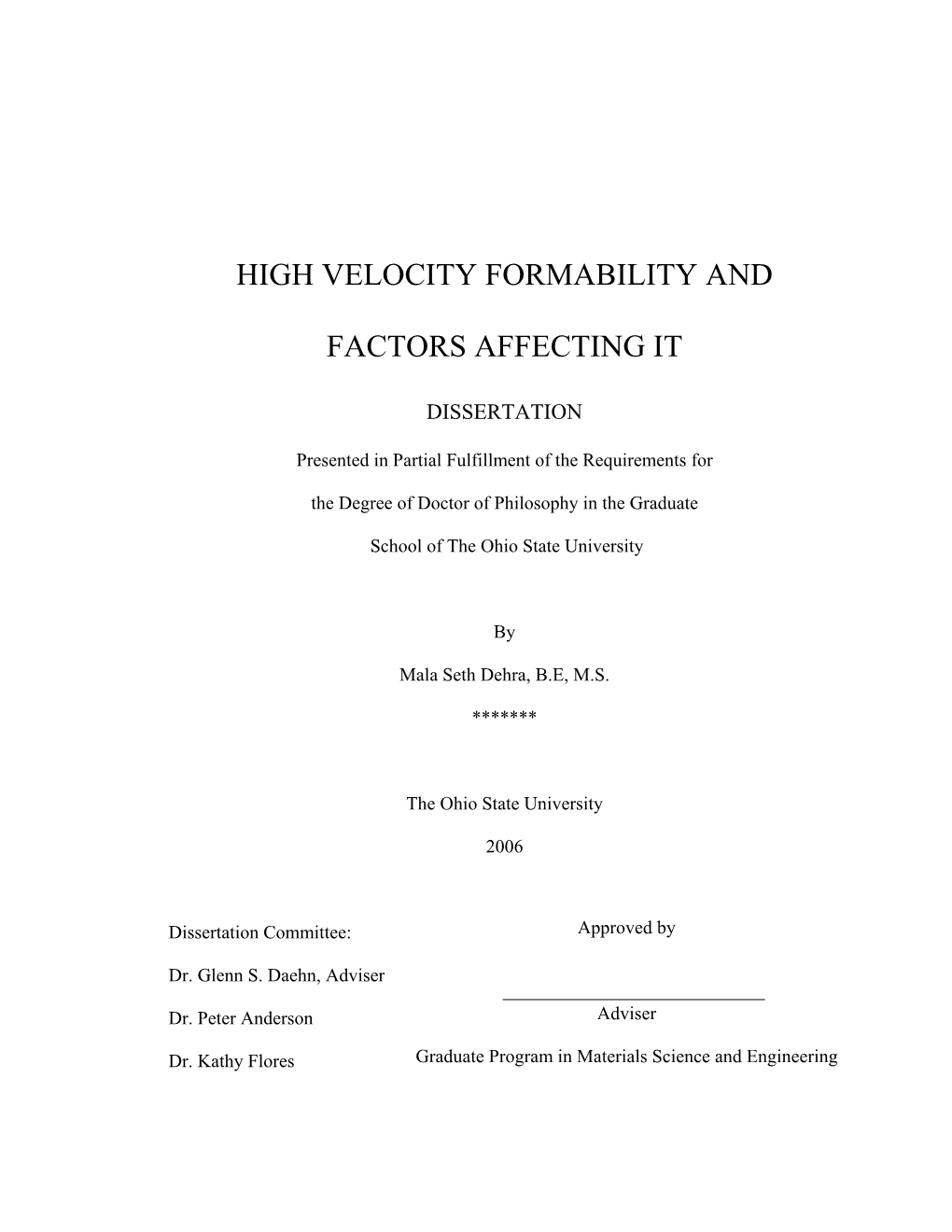
Load more
Recommended publications
-
Modification in Forming Die to Overcome Manufacturing Process
International Journal of Scientific & Engineering Research Volume 11, Issue 7, July-2020 ISSN 2229-5518 41 Modification in Forming Die to Overcome Manufacturing Process Limitation Prof.B.R.Chaudhari[1], PrathmeshKulkarni[2], Tejas Potdar[3], Omkar Pawar[4], Akhilesh Nikam[5] Abstract—Forming of sheet metal is common and vital process in manufacturing industry. Sheet metal forming is the plastic deformation of the work over an axis, creating a change in the parts geometry. Generally, there are two parts used in forming process; one of the part is punch which performs the stretching, bending and blanking operation and another is Die block which secularly clamps the workpiece and same operation as punch. Forming processes are particular manufacturing processes which make use of suitable stresses like compression, tension, shear, combined stresses which causes plastic deformation of the material to produce required shapes. During Forming process, no material is removed i.e. they are deformed and displaced. Some examples of forming processes are Forging, Sheet metal working, thread rolling, Electromagnetic forming, Explosive forming, rotary swaging, etc. Here the problem statement of the project is to combine these two parts design in one forming die which is now manufacturing separately on two different forming dies. Index Terms—Forming Die, Die Design, Blanking Process, Importance of Material Selection; ———————————————————— 1 INTRODUCTION heet metal is simply metal formed into thin and flat pieces. B. Plastic Deformation process: Bending, twisting, curling, S It is one of the fundamental forms used in metal forming deep drawing, necking, ribbing, seaming. can be cut and bent into variety of different shapes. -
10Th Int'l LS-DYNA® Users Conf
10th International LS-DYNA® Users Conference Metal Forming (3) Comparison Between Experimental and Numerical Results of Electromagnetic Forming Processes José Imbert*, Pierre L’eplattenier** and Michael Worswick* *Department of Mechanical and Mechatronics Engineering University of Waterloo, Waterloo, Ontario, Canada **LSTC, Livermore, California, USA Abstract Electromagnetic Forming (EMF) is a high speed metal forming process that is being studied with interest in both academia and industry as a way of improving existing sheet metal forming. The main thrust of the published research has been increasing the formability of aluminum alloys. Observing and measuring EMF process is made very difficult by the high speeds involved and the tooling used to obtain the final shapes. As with many other processes, numerical simulations can potentially be used to study the details of EMF; however, one limiting factor is the difficulty in modeling the process, since accurate models of both the structural and electromagnetic phenomena must be solved. Researchers have relied on simplifications that use analytical magnetic force distributions, on separate electromagnetic (EM) and structural codes or on in-house codes that can solve both problems simultaneously. In this paper, the predictive ability of the EM module of LS-DYNA® is assessed through a comparison between experimental and numerical results for samples formed by EMF. V-Channel and conical shaped samples were formed using two different EMF apparatuses. For the V-Channel samples a double rectangular coil was used and for the conical samples a spiral coil was used. Both processes were modeled using LS-DYNA®’s EM module. A comparison of the final experimental and numerical final shape and current profiles is presented. -
Advanced Manufacturing Processes Prof. Dr. Apurbba Kumar Sharma Department of Mechanical and Industrial Engineering Indian Institute of Technology, Roorkee
Advanced Manufacturing Processes Prof. Dr. Apurbba Kumar Sharma Department of Mechanical and Industrial Engineering Indian Institute of Technology, Roorkee Module - 5 Other Advanced Processes Lecture - 1 High Energy Rate Forming Processes Welcome to this new module on some new specialized material processing methods under the course advanced manufacturing processes. In the module 4, we have discussed about many advanced welding processes like solid state welding processes, laser welding processes, plasma arc welding processes etcetera. We have seen the working principles, advantages, limitations and applications of these processes. Moving on, let us see some new processes like high energy rate forming process, then rapid prototyping process and microwave material processing techniques. In the first of these techniques, let us consider high energy rate forming process. (Refer Slide Time: 01:50) The principle of these processes is like this. A number of methods have been developed to form metals through the application of large amounts of energy in a very short time interval. These processes are known as high energy rate forming processes. (Refer Slide Time: 02:13) Many metals tend to deform more rapidly under the ultra rapid load application rates used in these processes. Principle of high energy rate metal forming can be explained like this, if we have a ductile material, then this material can be deformed by working on it at a very faster speed and against a die of the shape required, the material can be shaped under the pressure of this applied energy. (Refer Slide Time: 02:57) Say for example, we have a sheet of metal which is of ductile in nature. -
(12) United States Patent 10
US007954357B2 (12) United States Patent (10) Patent N0.: US 7,954,357 B2 Bradley et al. (45) Date of Patent: Jun. 7, 2011 (54) DRIVER PLATE FOR ELECTROMAGNETIC (56) References Cited FORMING OF SHEET METAL U.S. PATENT DOCUMENTS (75) Inventors: John R. Bradley, Clarkston, MI (U S); 3,618,350 A 11/1971 Larrimer, Jr. et a1. Glenn S. Daehn, Columbus, OH (US) 7,069,756 B2 7/2006 Daehn 7,076,981 B2 7/2006 Bradley et al. (73) Assignees‘ GM Global Technology Operations 7,550,189 B1 * 6/2009 McKnight et a1‘ """"""" " 72/59 LLC, Detroit, MI (US); The Ohio State OTHER PUBLICATIONS University Research Foundation, W.H. Larrimer, Jr. and D.L. Duncan, “Transpactor-A Reusable Elec Columbus, OH (US) tromagnetic Forming T001 . ”, Technical Paper, 1973, pp. 1-14, Society of Manufacturing Engineers, Dearborn, MI. ( * ) Notice: Subject to any disclaimer, the term of this * Cited b examiner patent is extended or adjusted under 35 y U-S-C- 154(1)) by 837 days- Primary Examiner * Teresa M Ekiert (74) Attorney, Agent, or Firm * Reising Ethington RC. (21) App1.N0.: 11/867,734 (57) ABSTRACT (22) Filed; Oct 5, 2007 A multi-layer driver plate is disclosed for use in electromag netic sheet metal forming operations. In one embodiment, the (65) Prior Publication Data driver plate comprises a ?rst layer characterized by loW elec trical resistivity and thickness for inducement and application Us 2009/0090162 A1 APT- 9: 2009 of a suitable electromagnetic forming force, a second layer comprising an elastomeric material for compressing a sheet (51) Int_ CL metal workpiece against a die surface and then regaining its B21D 26/00 (200601) original pre-forming structure, and a third layer interposed (52) us. -
Electromagnetic Forming Processes: Material Behaviour and Computational Modelling François Bay, Anne-Claire Jeanson, José-Rodolfo Alves Zapata
Electromagnetic Forming Processes: Material Behaviour and Computational Modelling François Bay, Anne-Claire Jeanson, José-Rodolfo Alves Zapata To cite this version: François Bay, Anne-Claire Jeanson, José-Rodolfo Alves Zapata. Electromagnetic Forming Processes: Material Behaviour and Computational Modelling. 11th International Conference on Technology of Plasticity, ICTP 2014, Oct 2014, Nagoya, Japan. pp.793-800, 10.1016/j.proeng.2014.10.078. hal- 01221105 HAL Id: hal-01221105 https://hal-mines-paristech.archives-ouvertes.fr/hal-01221105 Submitted on 27 Oct 2015 HAL is a multi-disciplinary open access L’archive ouverte pluridisciplinaire HAL, est archive for the deposit and dissemination of sci- destinée au dépôt et à la diffusion de documents entific research documents, whether they are pub- scientifiques de niveau recherche, publiés ou non, lished or not. The documents may come from émanant des établissements d’enseignement et de teaching and research institutions in France or recherche français ou étrangers, des laboratoires abroad, or from public or private research centers. publics ou privés. Available online at www.sciencedirect.com ScienceDirect Procedia Engineering 81 ( 2014 ) 793 – 800 11th International Conference on Technology of Plasticity, ICTP 2014, 19-24 October 2014, Nagoya Congress Center, Nagoya, Japan Electromagnetic forming processes: material behaviour and computational modelling François Baya , Anne-Claire Jeansona,b, Jose Alves Zapataa aCenter for Material Forming (CEMEF) Mines Paristech – UMR CNRS 7635 BP 207, F-06904 Sophia-Antipolis cedex, France bI-Cube Research, 30 Boulevard de Thibaud, F-31100 Toulouse, France Abstract Electromagnetic Forming is a very promising high-speed forming process. However, designing these processes remains quite intricate as it leads to deal with strongly coupled multiphysics process and thus requires the use of computational models. -
Pretoria, 18 April 2007 2 No
Pretoria, 18 April 2007 2 No. 29790 GOVERNMENT GAZETTE. 18 APRIL 2007 CONTENTS Page Gazette No. No. No. GENERAL NOnCE Safety and Security, Department of General Notice 433 Explosives Act (15/2003): Draft Explosives Regulations, 2007 .. 3 29790 STAATSKOERANT, 18 APRIL 2007 NO.29790 3 GENERAL NOTICE NOTICE 433 OF 2007 DRAFT EXPLOSIVE REGULATIONS 2007 The Minister for Safety and Security intends to put the Explosives Act, 2003, (Act No. 15 of 2003) in operation once the Explosive Regulations has been finalised. Draft Regulations are hereby published for general information and comment from interested parties. IMPORTANT NOTE: This is merely a working document which is used to obtain the input of interest groups. The finalisation of the draft Regulations, will ultimately be done after the consultation process has been concluded. NO PART OF THE CONTENT OF THIS DOCUMENT OR ANY ALTERATION THEREOF MAY BE CONSIDERED AS A COMMITMENT TO THE FINAL PROVISIONS OF THE REGULATIONS Kindly note that as this is a working document certain technical correction with regard to the numbering, spacing and general layout still need to be done. Any comments, contributions or proposals on the Regulations may be submitted within 6 weeks from the date of publication of this Notice in writing to the following; e-mail address:[email protected] fax number: (012) 393 7126 Postal address: Dir. K Strydom Legal Service SAPS Private Bag X302 PRETORIA 0001 4 No. 29790 GOVERNMENT GAZETTE, 18 APRIL 2007 DRAFT EXPLOSIVES REGULATIONS Issued in terms of section 33(1) of the Explosives Act, 2003 (Act No 15 of 2003) 2007-02-28 STAATSKOERANT, 18 APRIL 2007 No.29790 5 DEPARTMENT OF SAFETY AND SECURITY Explosives Act, 2003 (Act No. -
First Results of Srf Cavity Fabrication by Electro-Hydraulic Forming at Cern S
THAA05 Proceedings of SRF2015, Whistler, BC, Canada FIRST RESULTS OF SRF CAVITY FABRICATION BY ELECTRO-HYDRAULIC FORMING AT CERN S. Atieh, A. Amorim Carvalho, I. Aviles Santillana, F. Bertinelli, R. Calaga, O. Capatina, G. Favre, M. Garlasché, F. Gerigk, S.A.E. Langeslag, K.M. Schirm, N. Valverde Alonso, CERN, Geneva, Switzerland G. Avrillaud, D. Alleman, J. Bonafe, J. Fuzeau, E. Mandel, P. Marty, A. Nottebaert, H. Peronnet, R. Plaut Bmax, Toulouse, France Abstract and combination of techniques: spinning, deep drawing, necking and hydroforming. In the framework of many accelerator projects relying on RF superconducting technology, shape conformity and processing time are key aspects for the optimization of niobium cavity fabrication. An alternative technique to traditional shaping methods, such as deep-drawing and spinning, is Electro-Hydraulic Forming (EHF). In EHF, cavities are obtained through ultra-high-speed deformation of blank sheets, using shockwaves induced in water by a pulsed electrical discharge. With respect to traditional methods, such a highly dynamic process can yield valuable results in terms of effectiveness, Figure 1: SPL 704 MHz elliptical cavity. repeatability, final shape precision, higher formability and reduced spring-back. In this paper, the first results of EHF on copper prototypes and ongoing developments for niobium for the Superconducting Proton Linac studies at CERN are discussed. The simulations performed in order to master the embedded multi-physics phenomena and to steer process parameters are also presented. INTRODUCTION Several projects at CERN require developments of new or spare superconducting RF cavities, in particular for the LHC consolidation, the LHC High Luminosity upgrade (HL-LHC), the Superconducting Proton Linac (SPL) and the Future Circular Collider (FCC) studies. -
Factors Effecting Electromagnetic Flat Sheet Forming Using the Uniform Pressure Coil
FACTORS EFFECTING ELECTROMAGNETIC FLAT SHEET FORMING USING THE UNIFORM PRESSURE COIL A THESIS Presented in Partial Fulfillment of the Requirements for the Degree of Master of Science in the Graduate School of The Ohio State University By Kristin E. Banik, B.S. ***** The Ohio State University 2008 Master’s Examination Committee: Approved by Professor Glenn S. Daehn, Adviser Professor Kathy Flores _______________________________ Adviser Graduate Program in Materials Science and Engineering ABSTRACT Electromagnetic forming is a possible alternative to sheet metal stamping. There are multiple limitations to the incumbent stamping methods including: complex alignment, changes to component shapes, and ductility issues, which often limits available formed geometry. Electromagnetic forming allows for the avoidance of some of these issues, but introduces a few other issues. In this thesis, the issues with electromagnetic forming will be discussed in conjunction with the application of the uniform pressure coil. Also, the effects on properties of the electromagnetically formed samples in comparison to the traditional samples will be presented. These properties include hardness, formability and interface issues. Lastly, discussed in this paper is the implementation of the Photon Doppler Velocimetry (PDV) system, a velocity measurement system used to determine the velocity of the workpiece and compare it to physics-based models of the process. ii Dedicated to my parents. iii ACKNOWLEDGMENTS I want to thank my adviser, Dr. Glenn Daehn for his support and guidance throughout graduate school and in running experiments and writing my thesis. I would also like to thank John Bradley, Steve Hatkevich and Allen Jones for their support of the work in our group as well as electromagnetic forming. -
Mechanical and Electrohydraulic Forming Processes
Process reliability and reproducibility of pneumo- mechanical and electrohydraulic forming processes W. Homberg1, E. Djakow1, O. Damerow1 1 Chair of Manufacturing and Forming Technology (LUF), Paderborn University, Paderborn Germany Abstract A sufficiently high process reliability and reproducibility is mandatory if a high-speed forming process is to be used in industrial production. A great deal of basic research work into pneumo-mechanical and electrohydraulic forming has been successfully performed in different institutions in the past. There, the focus has been more on process related correlations, such as the influence and interaction of different parameters on the course and result of those processes. The aspects of reliability and reproducibility have not been examined to a sufficient extent. Hence, in the case of pneumo-mechanical forming, insufficient investigations have been conducted into the effect that key parameters like the kinetic energy level, the filling height of the working media or the conditions inside the acceleration tube have on the reproducibility and course of the process. For electrohydraulic forming, the repeatability has worsened on occasions up to now. To improve the forming results and, in particular, the reputability of the process, it is necessary to examine the tool parameters associated with the electrodes and the working media. That is why research of this type is currently ongoing at the LUF. One important issue here is examining the options that exist for visualising the way the spark takes hold in the discharge chamber. Keywords High Speed Forming, Pneumo-mechanical Forming, Electrohydraulic Forming 1 Introduction The global trend towards the sustainable use of rare resources and the increasing demands of consumers for security items for products, for example, are leading to more complex components that are subject to high quality requirements. -
No 49, 1 May 1980, 1269
No. 49 1269 THE NEW ZEALAND GAZETTE Published by Authority WELLINGTON: THURSDAY, 1 MAY 1980 Revoking a Warrant Declaring an Area of Land in the Nelson 4. Paragraph 5 of the principal order is hereby amended Acclimatisation District to be a Wildlife Refuge by deleting the expression "$4.10 per acre" and substituting the expression "$10.15 per hectare". KEITH HOLYO AKE, Governor-General 5. Paragraph 6 of the principal order is hereby amended A PROCLAMATION by deleting the expression "$2.30 per acre-foot" and substi PURSUANT to section 14 of the Wildlife Act 1953, I, The tuting the expression '"$1.85 per 1,000 cubic metres". Right Honourable Sir Keith Jacka Holyoake, the Governor 6. The Third Schedule to the principal order is hereby General of New Zealand, hereby revoke the Warrant published amended by deleting those items following the item relating on 14 March 1963* notifying and declaring an area of land to the fifth season and substituting the following: to be a Wildlife Refuge. $ Given under the hand of His Excellency the Governor General, and issued under the Seal of New Zealand, Sixth season 6.09 per hectare. this 17th day of April 1980. Seventh season 7.11 per hectare. Eighth season 8.12 per hectare. D. A. HIGHET, Minister of Internal Affairs. Ninth season 9.14 per hectare. [L.S.] Goo SAVE THE QUEEN! Tenth season 10.15 per 'hectare. *New Zealand Gazette, No. 16 at page 331 P. G. MILLEN, Clerk of the Executive Council. (Wil. 34/10/5) (P.W. 64/7/1/15; D.O. -
Explosive Forming – Economical Technology for Aerospace Structures
View metadata, citation and similar papers at core.ac.uk brought to you by CORE provided by Directory of Open Access Journals EXPLOSIVE FORMING – ECONOMICAL TECHNOLOGY FOR AEROSPACE STRUCTURES Anniversary Session “Celebrating 100 year of the first jet aircraft invented by Henri Coanda”, organized by INCAS, COMOTI and Henri Coanda Association, 14 December 2010, Bucharest, Romania Victor GHIZDAVU*, Niculae MARIN** *Corresponding author Military Technical Academy of Bucharest, Bdul George Coşbuc 81-83, Sector 5, 050141, Romania [email protected] **Aerospace Consulting Bdul Iuliu Maniu 220, Bucharest 061126, Romania [email protected] DOI: 10.13111/2066-8201.2010.2.4.15 Abstract: The explosive forming represents a technological alternative for obtaining small-lot parts, with inexpensive and efficient manufacturing preparation. The explosive forming processing methods present a series of important advantages, being recommended for the wide-scale application in the aerospace industry. The economic benefit varies from case to case, independent from the part type, manufacturing series and user. Key Words: explosive forming, economical technology, wide-scale application, aerospace structures. 1. INTRODUCTION The explosive forming processing methods present a series of important advantages, being recommended for the wide-scale application in the aerospace industry. By using the high explosive forming, items can be obtained, with different shapes and almost unlimited overall size, made of heavy-duty materials. Economically, there is the possibility of costs decreasing of the forming operation, below 1/10th of those necessary for classic forming technologies, especially in what concerns the prototype and small-scale production. The economic benefit varies from case to case, independent from the part type, manufacturing series and user. -
31St Daaam International Symposium on Intelligent Manufacturing and Automation
31ST DAAAM INTERNATIONAL SYMPOSIUM ON INTELLIGENT MANUFACTURING AND AUTOMATION DOI: 10.2507/31st.daaam.proceedings.055 TECHNOLOGIES OF HIGH-VELOCITY FORMING Darko Sunjic, Stipo Buljan & E. Gomes This Publication has to be referred as: Sunjic, D[arko]; Buljan, S[tipo] & Gomes, E[duarda] (2020). Technologies of High-Velocity Forming, Proceedings of the 31st DAAAM International Symposium, pp.0403-0407, B. Katalinic (Ed.), Published by DAAAM International, ISBN 978-3-902734-29-7, ISSN 1726-9679, Vienna, Austria DOI: 10.2507/31st.daaam.proceedings.055 Abstract Technologies of high-velocity forming are mostly used for forming workpieces in which conventional technologies do not have a good effect. It is not possible to form certain workpieces with conventional technologies due to the complexity of the surfaces and shapes, type of materials, dimensions of the workpiece or other technical parameters of the workpiece. Increased market demands, especially in the automotive, aerospace and military industries, have expedited the development of new technologies and thus high-velocity technologies. Some of the technologies which are included in this are explosive forming, electromagnetic forming, electrohydraulic forming, and others. The paper describes these technologies and gives their advantages and disadvantages. Keywords: unconventional technologies; explosive forming; electromagnetic forming; high-velocity forming; forming 1. Introduction The paper describes the technologies that are best known and the technologies that are most used in high-velocity forming. High-velocity forming or high energy rate forming include technologies that use a large amount of energy in a very short time interval. The reason for these needs as well as the division itself are explained in the paper.