Calpain 9 Functions in TNF Receptor Mediated Apoptosis
Total Page:16
File Type:pdf, Size:1020Kb
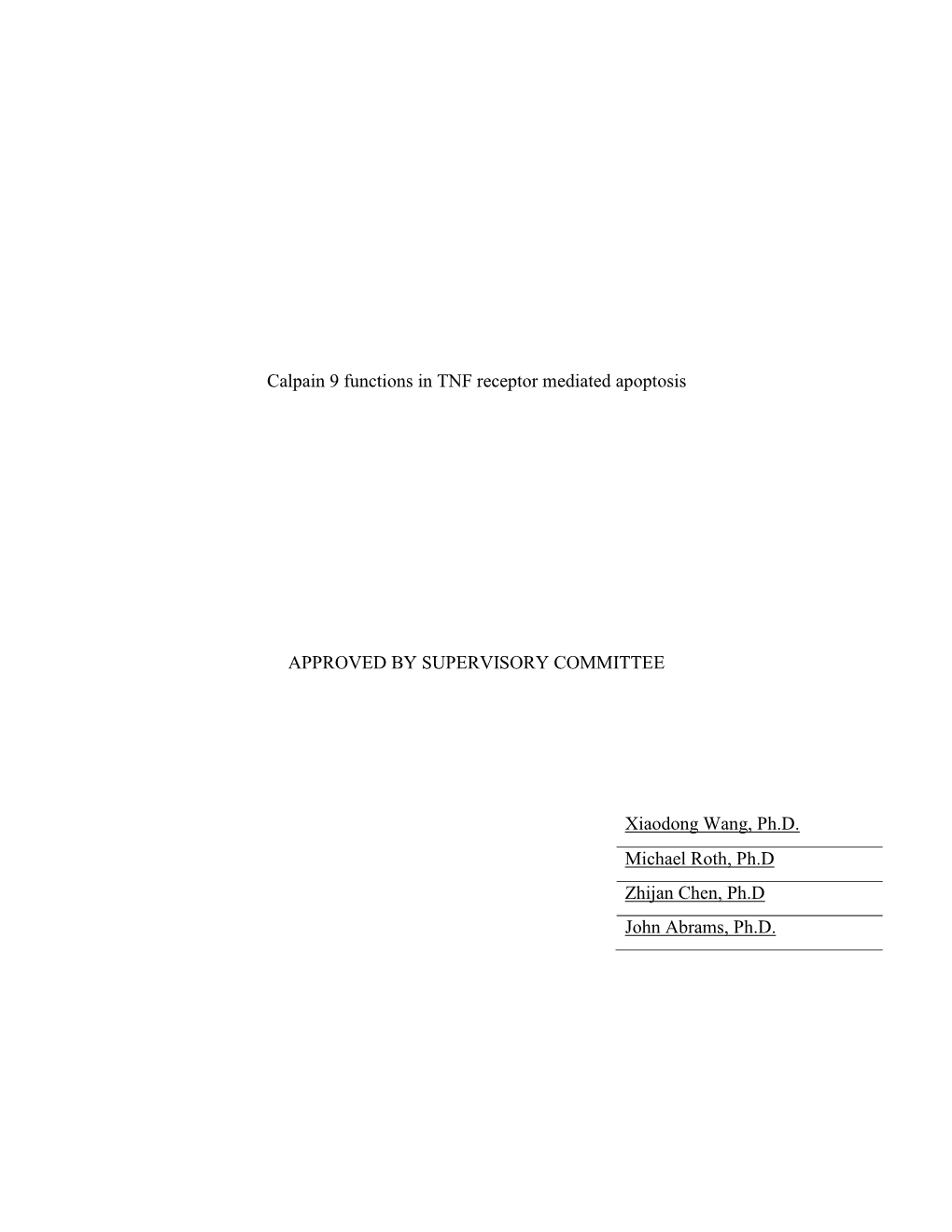
Load more
Recommended publications
-
Universidade Estadual De Campinas Instituto De Biologia
UNIVERSIDADE ESTADUAL DE CAMPINAS INSTITUTO DE BIOLOGIA VERÔNICA APARECIDA MONTEIRO SAIA CEREDA O PROTEOMA DO CORPO CALOSO DA ESQUIZOFRENIA THE PROTEOME OF THE CORPUS CALLOSUM IN SCHIZOPHRENIA CAMPINAS 2016 1 VERÔNICA APARECIDA MONTEIRO SAIA CEREDA O PROTEOMA DO CORPO CALOSO DA ESQUIZOFRENIA THE PROTEOME OF THE CORPUS CALLOSUM IN SCHIZOPHRENIA Dissertação apresentada ao Instituto de Biologia da Universidade Estadual de Campinas como parte dos requisitos exigidos para a obtenção do Título de Mestra em Biologia Funcional e Molecular na área de concentração de Bioquímica. Dissertation presented to the Institute of Biology of the University of Campinas in partial fulfillment of the requirements for the degree of Master in Functional and Molecular Biology, in the area of Biochemistry. ESTE ARQUIVO DIGITAL CORRESPONDE À VERSÃO FINAL DA DISSERTAÇÃO DEFENDIDA PELA ALUNA VERÔNICA APARECIDA MONTEIRO SAIA CEREDA E ORIENTADA PELO DANIEL MARTINS-DE-SOUZA. Orientador: Daniel Martins-de-Souza CAMPINAS 2016 2 Agência(s) de fomento e nº(s) de processo(s): CNPq, 151787/2F2014-0 Ficha catalográfica Universidade Estadual de Campinas Biblioteca do Instituto de Biologia Mara Janaina de Oliveira - CRB 8/6972 Saia-Cereda, Verônica Aparecida Monteiro, 1988- Sa21p O proteoma do corpo caloso da esquizofrenia / Verônica Aparecida Monteiro Saia Cereda. – Campinas, SP : [s.n.], 2016. Orientador: Daniel Martins de Souza. Dissertação (mestrado) – Universidade Estadual de Campinas, Instituto de Biologia. 1. Esquizofrenia. 2. Espectrometria de massas. 3. Corpo caloso. -
Calpain-5 Gene Expression in the Mouse Eye and Brain
Schaefer et al. BMC Res Notes (2017) 10:602 DOI 10.1186/s13104-017-2927-8 BMC Research Notes RESEARCH NOTE Open Access Calpain‑5 gene expression in the mouse eye and brain Kellie Schaefer1, MaryAnn Mahajan1, Anuradha Gore1, Stephen H. Tsang3, Alexander G. Bassuk4 and Vinit B. Mahajan1,2* Abstract Objective: Our objective was to characterize CAPN5 gene expression in the mouse central nervous system. Mouse brain and eye sections were probed with two high-afnity RNA oligonucleotide analogs designed to bind CAPN5 RNA and one scramble, control oligonucleotide. Images were captured in brightfeld. Results: CAPN5 RNA probes were validated on mouse breast cancer tumor tissue. In the eye, CAPN5 was expressed in the ganglion cell, inner nuclear and outer nuclear layers of the retina. Signal could not be detected in the ciliary body or the iris because of the high density of melanin. In the brain, CAPN5 was expressed in the granule cell layers of the hippocampus and cerebellum. There was scattered expression in pons. The visual cortex showed faint signal. Most signal in the brain was in a punctate pattern. Keywords: CAPN5, Calpain, In situ hybridization, Retina, Brain, Gene expression Introduction pigmentosa, retinal neovascularization, and proliferative Calpain-5 (CAPN5) is a member of the calpain family of retinopathy. Which ultimately leads to blindness [20]. calcium-activated proteases that target a variety of path- Currently there is no treatment. ways to exert control over numerous processes, includ- An important question to understanding how CAPN5 ing tissue necrosis, cytoskeletal remodeling, cell-cycle leads to disease is identifying which tissues CAPN5 is control, cell migration, myofbril turnover, regulation expressed in and the levels of CAPN5 in those tissues. -
Reconstructability Analysis As a Tool for Identifying Gene-Gene Interactions in Studies of Human Diseases
Portland State University PDXScholar Systems Science Faculty Publications and Presentations Systems Science 3-2010 Reconstructability Analysis As A Tool For Identifying Gene-Gene Interactions In Studies Of Human Diseases Stephen Shervais Eastern Washington University Patricia L. Kramer Oregon Health & Science University Shawn K. Westaway Oregon Health & Science University Nancy J. Cox University of Chicago Martin Zwick Portland State University, [email protected] Follow this and additional works at: https://pdxscholar.library.pdx.edu/sysc_fac Part of the Bioinformatics Commons, Diseases Commons, and the Genomics Commons Let us know how access to this document benefits ou.y Citation Details Shervais, S., Kramer, P. L., Westaway, S. K., Cox, N. J., & Zwick, M. (2010). Reconstructability Analysis as a Tool for Identifying Gene-Gene Interactions in Studies of Human Diseases. Statistical Applications In Genetics & Molecular Biology, 9(1), 1-25. This Article is brought to you for free and open access. It has been accepted for inclusion in Systems Science Faculty Publications and Presentations by an authorized administrator of PDXScholar. Please contact us if we can make this document more accessible: [email protected]. Statistical Applications in Genetics and Molecular Biology Volume 9, Issue 1 2010 Article 18 Reconstructability Analysis as a Tool for Identifying Gene-Gene Interactions in Studies of Human Diseases Stephen Shervais∗ Patricia L. Kramery Shawn K. Westawayz Nancy J. Cox∗∗ Martin Zwickyy ∗Eastern Washington University, [email protected] yOregon Health & Science University, [email protected] zOregon Health & Science University, [email protected] ∗∗University of Chicago, [email protected] yyPortland State University, [email protected] Copyright c 2010 The Berkeley Electronic Press. -
Propranolol-Mediated Attenuation of MMP-9 Excretion in Infants with Hemangiomas
Supplementary Online Content Thaivalappil S, Bauman N, Saieg A, Movius E, Brown KJ, Preciado D. Propranolol-mediated attenuation of MMP-9 excretion in infants with hemangiomas. JAMA Otolaryngol Head Neck Surg. doi:10.1001/jamaoto.2013.4773 eTable. List of All of the Proteins Identified by Proteomics This supplementary material has been provided by the authors to give readers additional information about their work. © 2013 American Medical Association. All rights reserved. Downloaded From: https://jamanetwork.com/ on 10/01/2021 eTable. List of All of the Proteins Identified by Proteomics Protein Name Prop 12 mo/4 Pred 12 mo/4 Δ Prop to Pred mo mo Myeloperoxidase OS=Homo sapiens GN=MPO 26.00 143.00 ‐117.00 Lactotransferrin OS=Homo sapiens GN=LTF 114.00 205.50 ‐91.50 Matrix metalloproteinase‐9 OS=Homo sapiens GN=MMP9 5.00 36.00 ‐31.00 Neutrophil elastase OS=Homo sapiens GN=ELANE 24.00 48.00 ‐24.00 Bleomycin hydrolase OS=Homo sapiens GN=BLMH 3.00 25.00 ‐22.00 CAP7_HUMAN Azurocidin OS=Homo sapiens GN=AZU1 PE=1 SV=3 4.00 26.00 ‐22.00 S10A8_HUMAN Protein S100‐A8 OS=Homo sapiens GN=S100A8 PE=1 14.67 30.50 ‐15.83 SV=1 IL1F9_HUMAN Interleukin‐1 family member 9 OS=Homo sapiens 1.00 15.00 ‐14.00 GN=IL1F9 PE=1 SV=1 MUC5B_HUMAN Mucin‐5B OS=Homo sapiens GN=MUC5B PE=1 SV=3 2.00 14.00 ‐12.00 MUC4_HUMAN Mucin‐4 OS=Homo sapiens GN=MUC4 PE=1 SV=3 1.00 12.00 ‐11.00 HRG_HUMAN Histidine‐rich glycoprotein OS=Homo sapiens GN=HRG 1.00 12.00 ‐11.00 PE=1 SV=1 TKT_HUMAN Transketolase OS=Homo sapiens GN=TKT PE=1 SV=3 17.00 28.00 ‐11.00 CATG_HUMAN Cathepsin G OS=Homo -
Discovery of Endoplasmic Reticulum Calcium Stabilizers to Rescue ER-Stressed Podocytes in Nephrotic Syndrome
Discovery of endoplasmic reticulum calcium stabilizers to rescue ER-stressed podocytes in nephrotic syndrome Sun-Ji Parka, Yeawon Kima, Shyh-Ming Yangb, Mark J. Hendersonb, Wei Yangc, Maria Lindahld, Fumihiko Uranoe, and Ying Maggie Chena,1 aDivision of Nephrology, Department of Medicine, Washington University School of Medicine, St. Louis, MO 63110; bNational Center for Advancing Translational Sciences, National Institutes of Health, Rockville, MD 20850; cDepartment of Genetics, Washington University School of Medicine, St. Louis, MO 63110; dInstitute of Biotechnology, University of Helsinki, Helsinki, Finland 00014; and eDivision of Endocrinology, Metabolism, and Lipid Research, Department of Medicine, Washington University School of Medicine, St. Louis, MO 63110 Edited by Martin R. Pollak, Beth Israel Deaconess Medical Center, Brookline, MA, and approved May 28, 2019 (received for review August 16, 2018) Emerging evidence has established primary nephrotic syndrome activating transcription factor 6 (ATF6), which act as proximal (NS), including focal segmental glomerulosclerosis (FSGS), as a sensors of ER stress. ER stress activates these sensors by inducing primary podocytopathy. Despite the underlying importance of phosphorylation and homodimerization of IRE1α and PERK/ podocyte endoplasmic reticulum (ER) stress in the pathogenesis of eukaryotic initiation factor 2α (eIF2α), as well as relocalization of NS, no treatment currently targets the podocyte ER. In our mono- ATF6 to the Golgi, where it is cleaved by S1P/S2P proteases from genic podocyte ER stress-induced NS/FSGS mouse model, the 90 kDa to the active 50-kDa ATF6 (8), leading to activation of podocyte type 2 ryanodine receptor (RyR2)/calcium release channel their respective downstream transcription factors, spliced XBP1 on the ER was phosphorylated, resulting in ER calcium leak and (XBP1s), ATF4, and p50ATF6 (8–10). -
Characterization of the Goose CAPN3 Gene and Its Expression Pattern in Muscle Tissues of Sichuan White Geese at Different Growth Stages
http://www.jstage.jst.go.jp/browse/jpsa doi:10.2141/ jpsa.0170150 Copyright Ⓒ 2018, Japan Poultry Science Association. Characterization of the Goose CAPN3 Gene and its Expression Pattern in Muscle Tissues of Sichuan White Geese at Different Growth Stages Hengyong Xu*, Yahui Zhang*, Quan Zou, Liang Li, Chunchun Han, Hehe Liu, Jiwei Hu, Tao Zhong and Yan Wang Farm Animal Genetic Resources Exploration and Innovation Key Laboratory of Sichuan Province, Sichuan Agricultural University, Chengdu 611130, China Calpain 3 (CAPN3), also known as p94, is associated with multiple production traits in domestic animals. However, the molecular characteristics of the CAPN3 gene and its expression profile in goose tissues have not been reported. In this study, CAPN3 cDNA of the Sichuan white goose was cloned, sequenced, and characterized. The CAPN3 full-length cDNA sequence consists of a 2,316-bp coding sequence (CDS) that encodes 771 amino acids with a molecular mass of 89,019 kDa. The protein was predicted to have no signal peptide, but several N-glycosylation, O- glycosylation, and phosphorylation sites. The secondary structure of CAPN3 was predicted to be 38.65% α-helical. Sequence alignment showed that CAPN3 of Sichuan white goose shared more than 90% amino acid sequence similarity with those of Japanese quail, turkey, helmeted guineafowl, duck, pigeon, and chicken. Phylogenetic tree analysis showed that goose CAPN3 has a close genetic relationship and small evolutionary distance with those of the birds. qRT-PCR analysis showed that in 15-day-old animals, the expression level of CAPN3 was significantly higher in breast muscle than in thigh tissues. -
Evaluation of the Genetic Susceptibility to the Metabolic Syndrome by the CAPN10 SNP19 Gene in the Population of South Benin
International Journal of Molecular Biology: Open Access Research Article Open Access Evaluation of the genetic susceptibility to the metabolic syndrome by the CAPN10 SNP19 gene in the population of South Benin Abstract Volume 4 Issue 6 - 2019 Metabolic syndrome is a multifactorial disorder whose etiology is resulting from the Nicodème Worou Chabi,1,2 Basile G interaction between genetic and environmental factors. Calpain 10 (CAPN10) is the first Sognigbé,1 Esther Duéguénon,1 Véronique BT gene associated with type 2 diabetes that has been identified by positional cloning with 1 1 sequencing method. This gene codes for cysteine protease; ubiquitously expressed in all Tinéponanti, Arnaud N Kohonou, Victorien 2 1 tissues, it is involved in the fundamental physiopathological aspects of insulin resistance T Dougnon, Lamine Baba Moussa and insulin secretion of type 2 diabetes. The goal of this study was to evaluate the genetic 1Department of Biochemistry and Cell Biology, University of susceptibility to the metabolic syndrome by the CAPN10 gene in the population of southern Abomey-Calavi, Benin 2 Benin. This study involved apparently healthy individuals’ aged 18 to 80 in four ethnic Laboratory of Research in Applied Biology, Polytechnic School of Abomey-Calavi, University of Abomey-Calavi, Benin groups in southern Benin. It included 74 subjects with metabolic syndrome and 323 non- metabolic syndrome patients who served as controls, with 222 women versus 175 men Correspondence: Nicodème Worou Chabi, Laboratory with an average age of 40.58 ± 14.03 years old. All subjects were genotyped for the SNP of Biochemistry and Molecular Biology, Department of 19 polymorphism of the CAPN10 gene with the PCR method in order to find associations Biochemistry and Cell Biology, Faculty of Science and between this polymorphism and the metabolic syndrome. -
CCN3 and Calcium Signaling Alain Lombet1, Nathalie Planque2, Anne-Marie Bleau2, Chang Long Li2 and Bernard Perbal*2
Cell Communication and Signaling BioMed Central Review Open Access CCN3 and calcium signaling Alain Lombet1, Nathalie Planque2, Anne-Marie Bleau2, Chang Long Li2 and Bernard Perbal*2 Address: 1CNRS UMR 8078, Hôpital Marie Lannelongue, 133, Avenue de la Résistance 92350 Le PLESSIS-ROBINSON, France and 2Laboratoire d'Oncologie Virale et Moléculaire, Tour 54, Case 7048, Université Paris 7-D.Diderot, 2 Place Jussieu 75005 PARIS, France Email: Alain Lombet - [email protected]; Nathalie Planque - [email protected]; Anne-Marie Bleau - [email protected]; Chang Long Li - [email protected]; Bernard Perbal* - [email protected] * Corresponding author Published: 15 August 2003 Received: 26 June 2003 Accepted: 15 August 2003 Cell Communication and Signaling 2003, 1:1 This article is available from: http://www.biosignaling.com/content/1/1/1 © 2003 Lombet et al; licensee BioMed Central Ltd. This is an Open Access article: verbatim copying and redistribution of this article are permitted in all media for any purpose, provided this notice is preserved along with the article's original URL. Abstract The CCN family of genes consists presently of six members in human (CCN1-6) also known as Cyr61 (Cystein rich 61), CTGF (Connective Tissue Growth Factor), NOV (Nephroblastoma Overexpressed gene), WISP-1, 2 and 3 (Wnt-1 Induced Secreted Proteins). Results obtained over the past decade have indicated that CCN proteins are matricellular proteins, which are involved in the regulation of various cellular functions, such as proliferation, differentiation, survival, adhesion and migration. The CCN proteins have recently emerged as regulatory factors involved in both internal and external cell signaling. -
Investigation of the Underlying Hub Genes and Molexular Pathogensis in Gastric Cancer by Integrated Bioinformatic Analyses
bioRxiv preprint doi: https://doi.org/10.1101/2020.12.20.423656; this version posted December 22, 2020. The copyright holder for this preprint (which was not certified by peer review) is the author/funder. All rights reserved. No reuse allowed without permission. Investigation of the underlying hub genes and molexular pathogensis in gastric cancer by integrated bioinformatic analyses Basavaraj Vastrad1, Chanabasayya Vastrad*2 1. Department of Biochemistry, Basaveshwar College of Pharmacy, Gadag, Karnataka 582103, India. 2. Biostatistics and Bioinformatics, Chanabasava Nilaya, Bharthinagar, Dharwad 580001, Karanataka, India. * Chanabasayya Vastrad [email protected] Ph: +919480073398 Chanabasava Nilaya, Bharthinagar, Dharwad 580001 , Karanataka, India bioRxiv preprint doi: https://doi.org/10.1101/2020.12.20.423656; this version posted December 22, 2020. The copyright holder for this preprint (which was not certified by peer review) is the author/funder. All rights reserved. No reuse allowed without permission. Abstract The high mortality rate of gastric cancer (GC) is in part due to the absence of initial disclosure of its biomarkers. The recognition of important genes associated in GC is therefore recommended to advance clinical prognosis, diagnosis and and treatment outcomes. The current investigation used the microarray dataset GSE113255 RNA seq data from the Gene Expression Omnibus database to diagnose differentially expressed genes (DEGs). Pathway and gene ontology enrichment analyses were performed, and a proteinprotein interaction network, modules, target genes - miRNA regulatory network and target genes - TF regulatory network were constructed and analyzed. Finally, validation of hub genes was performed. The 1008 DEGs identified consisted of 505 up regulated genes and 503 down regulated genes. -
Supplementary Table S4. FGA Co-Expressed Gene List in LUAD
Supplementary Table S4. FGA co-expressed gene list in LUAD tumors Symbol R Locus Description FGG 0.919 4q28 fibrinogen gamma chain FGL1 0.635 8p22 fibrinogen-like 1 SLC7A2 0.536 8p22 solute carrier family 7 (cationic amino acid transporter, y+ system), member 2 DUSP4 0.521 8p12-p11 dual specificity phosphatase 4 HAL 0.51 12q22-q24.1histidine ammonia-lyase PDE4D 0.499 5q12 phosphodiesterase 4D, cAMP-specific FURIN 0.497 15q26.1 furin (paired basic amino acid cleaving enzyme) CPS1 0.49 2q35 carbamoyl-phosphate synthase 1, mitochondrial TESC 0.478 12q24.22 tescalcin INHA 0.465 2q35 inhibin, alpha S100P 0.461 4p16 S100 calcium binding protein P VPS37A 0.447 8p22 vacuolar protein sorting 37 homolog A (S. cerevisiae) SLC16A14 0.447 2q36.3 solute carrier family 16, member 14 PPARGC1A 0.443 4p15.1 peroxisome proliferator-activated receptor gamma, coactivator 1 alpha SIK1 0.435 21q22.3 salt-inducible kinase 1 IRS2 0.434 13q34 insulin receptor substrate 2 RND1 0.433 12q12 Rho family GTPase 1 HGD 0.433 3q13.33 homogentisate 1,2-dioxygenase PTP4A1 0.432 6q12 protein tyrosine phosphatase type IVA, member 1 C8orf4 0.428 8p11.2 chromosome 8 open reading frame 4 DDC 0.427 7p12.2 dopa decarboxylase (aromatic L-amino acid decarboxylase) TACC2 0.427 10q26 transforming, acidic coiled-coil containing protein 2 MUC13 0.422 3q21.2 mucin 13, cell surface associated C5 0.412 9q33-q34 complement component 5 NR4A2 0.412 2q22-q23 nuclear receptor subfamily 4, group A, member 2 EYS 0.411 6q12 eyes shut homolog (Drosophila) GPX2 0.406 14q24.1 glutathione peroxidase -
Calpain-10 Regulates Actin Dynamics by Proteolysis of Microtubule-Associated Protein 1B
www.nature.com/scientificreports OPEN Calpain-10 regulates actin dynamics by proteolysis of microtubule-associated protein 1B Received: 15 September 2015 Tomohisa Hatta1, Shun-ichiro Iemura1,6, Tomokazu Ohishi2, Hiroshi Nakayama3, Accepted: 1 November 2018 Hiroyuki Seimiya 2, Takao Yasuda4, Katsumi Iizuka5, Mitsunori Fukuda4, Jun Takeda5, Published: xx xx xxxx Tohru Natsume1 & Yukio Horikawa5 Calpain-10 (CAPN10) is the calpain family protease identifed as the frst candidate susceptibility gene for type 2 diabetes mellitus (T2DM). However, the detailed molecular mechanism has not yet been elucidated. Here we report that CAPN10 processes microtubule associated protein 1 (MAP1) family proteins into heavy and light chains and regulates their binding activities to microtubules and actin flaments. Immunofuorescent analysis of Capn10−/− mouse embryonic fbroblasts shows that MAP1B, a member of the MAP1 family of proteins, is localized at actin flaments rather than at microtubules. Furthermore, fuorescence recovery after photo-bleaching analysis shows that calpain-10 regulates actin dynamics via MAP1B cleavage. Moreover, in pancreatic islets from CAPN10 knockout mice, insulin secretion was signifcantly increased both at the high and low glucose levels. These fndings indicate that defciency of calpain-10 expression may afect insulin secretion by abnormal actin reorganization, coordination and dynamics through MAP1 family processing. Calpains are a family of intracellular non-lysosomal calcium-activated neutral cysteine proteases known to cleave various substrate proteins and modulate their activities. Tere are 16 calpains, some of which are ubiquitously expressed and others displaying tissue-specifc distribution. Some calpains contain a penta-EF-hand domain (typical or classical calpains), and others do not (atypical calpains). Several calpain family members are impli- cated in the development of various diseases including Alzheimer’s disease, cataracts, ischemic stroke, traumatic brain injury, limb-girdle muscular dystrophy 2A and type 2 diabetes mellitus (T2DM)1. -
Proteomics of Serum Extracellular Vesicles Identifies a Novel COPD Biomarker, Fibulin-3 from Elastic Fibres
ORIGINAL ARTICLE COPD Proteomics of serum extracellular vesicles identifies a novel COPD biomarker, fibulin-3 from elastic fibres Taro Koba 1, Yoshito Takeda1, Ryohei Narumi2, Takashi Shiromizu2, Yosui Nojima 3, Mari Ito3, Muneyoshi Kuroyama1, Yu Futami1, Takayuki Takimoto4, Takanori Matsuki1, Ryuya Edahiro1, Satoshi Nojima5, Yoshitomo Hayama1, Kiyoharu Fukushima1, Haruhiko Hirata1, Shohei Koyama1, Kota Iwahori1, Izumi Nagatomo1, Mayumi Suzuki1, Yuya Shirai1, Teruaki Murakami1, Kaori Nakanishi 1, Takeshi Nakatani1, Yasuhiko Suga1, Kotaro Miyake1, Takayuki Shiroyama1, Hiroshi Kida 1, Takako Sasaki6, Koji Ueda7, Kenji Mizuguchi3, Jun Adachi2, Takeshi Tomonaga2 and Atsushi Kumanogoh1 ABSTRACT There is an unmet need for novel biomarkers in the diagnosis of multifactorial COPD. We applied next-generation proteomics to serum extracellular vesicles (EVs) to discover novel COPD biomarkers. EVs from 10 patients with COPD and six healthy controls were analysed by tandem mass tag-based non-targeted proteomics, and those from elastase-treated mouse models of emphysema were also analysed by non-targeted proteomics. For validation, EVs from 23 patients with COPD and 20 healthy controls were validated by targeted proteomics. Using non-targeted proteomics, we identified 406 proteins, 34 of which were significantly upregulated in patients with COPD. Of note, the EV protein signature from patients with COPD reflected inflammation and remodelling. We also identified 63 upregulated candidates from 1956 proteins by analysing EVs isolated from mouse models. Combining human and mouse biomarker candidates, we validated 45 proteins by targeted proteomics, selected reaction monitoring. Notably, levels of fibulin-3, tripeptidyl- peptidase 2, fibulin-1, and soluble scavenger receptor cysteine-rich domain-containing protein were significantly higher in patients with COPD.