John Patrick Stephen Mowat Phd Thesis
Total Page:16
File Type:pdf, Size:1020Kb
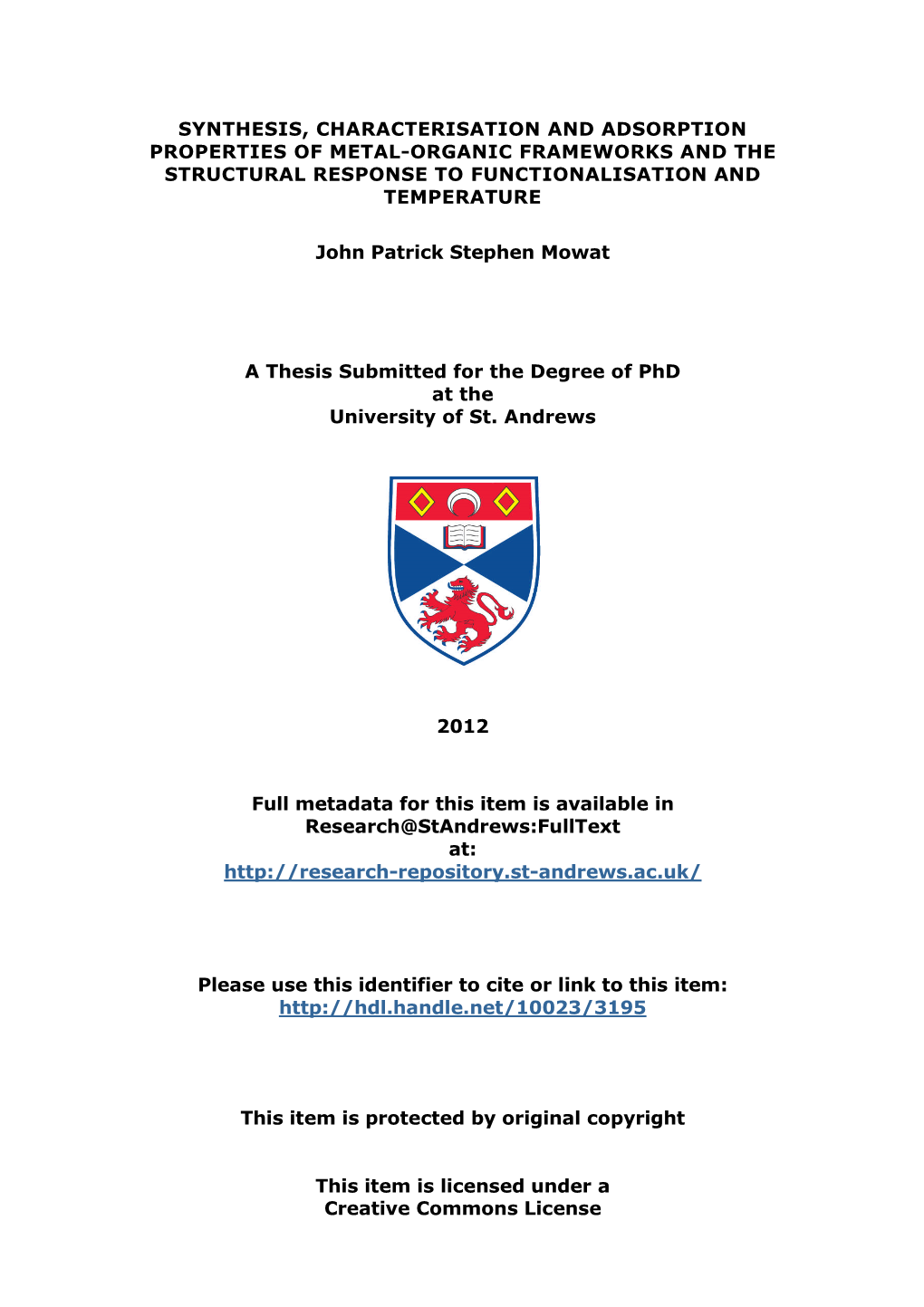
Load more
Recommended publications
-
IUPAC-NIST Solubility Data Series. 87. Rare Earth Metal Chlorides in Water and Aqueous Systems
IUPAC-NIST Solubility Data Series. 87. Rare Earth Metal Chlorides in Water and Aqueous Systems. Part 1. Scandium Group „Sc, Y, La… Tomasz Mioduskia… Institute of Nuclear Chemistry and Technology, Warsaw, Poland Cezary Gumińskib… Department of Chemistry, University of Warsaw, Warsaw, Poland Dewen Zengc… College of Chemistry and Chemical Engineering, Hunan University, Hunan, People’s Republic of China ͑Received 12 May 2008; revised manuscript received 20 May 2008; accepted 20 May 2008; published online 15 October 2008͒ This volume presents solubility data for rare earth metal chlorides in water and in ternary and quaternary aqueous systems. The material is divided into three parts: scan- dium group ͑Sc, Y, La͒, light lanthanide ͑Ce-Eu͒, and heavy lanthanide ͑Gd-Lu͒ chlo- rides; this part covers the scandium group. Compilations of all available experimental data are introduced for each rare earth metal chloride with a corresponding critical evalu- ation. Every such evaluation contains a tabulated collection of all solubility results in ͑ ͒ water, a scheme of the water-rich part of the equilibrium Y, La, Ln Cl3 –H2O phase diagram, solubility equation͑s͒, a selection of suggested solubility data, and a discussion of the multicomponent systems. Because the ternary and quaternary systems were almost never studied more than once, no critical evaluations or systematic comparisons of such data were possible. Only simple chlorides ͑no complexes͒ are treated as the input sub- stances in this work. The literature ͑including a thorough coverage of papers in Chinese and Russian͒ has been covered through the middle of 2007. © 2008 American Institute of Physics. ͓DOI: 10.1063/1.2956740͔ Key words: aqueous solution; lanthanum chloride; phase diagram; scandium chloride; solubility; yttrium chloride. -
Rights Reserved Organoscandium Chemistry
@ 1974 KARL DEE SMITH ALL RIGHTS RESERVED ORGANOSCANDIUM CHEMISTRY by KARL DEE SMITH A DISSERTATION Submitted in partial fulfillment of the requirements for the degree of Doctor of Philosophy in the Department of Chemistry in the Graduate School of The University of Alabama UNIVERSITY, AL1\BAMA 1973 --- e···· ACKNOWLEDGMENTS The author wishes to express his deep appreciation to: Dr. D. F. Smith and Dr. B. W. Ponder for their understanding, encouragement, and guidance throughout the course of this research. Steve Seale for his many hours spent in setting up a workable computer library to permit the completion of this work to become a reality. Merle Watson for his many services rendered in the making of the special glass apparatus needed throughout the course of this work. The computer operators, Steve Watson, Mike Webb, Bob McGwier, Al Martin, and Bill Gammon for their cooperation in efficiently running the hundreds of computer programs needed for the completion of this work. Sam Hassel, G. M. Nichols, and Harold Moore for their many services rendered in the maintenance, stockroom, and electronics fields, respectively. The secretaries for their services rendered. Segail Friedman for typing the final manuscript of this work. His wife, Becky, .and to Angela and Christopher for their confidence, encouragement, understanding, and love shown in every way. ii TABLE OF CONTENTS Page ACKNOWLEDGivIENTS • • ii LIS'l' OF TABLES . iv LIST OF FIGURES • vi Chapter I. INTRODUCTION 1 II. EXPERIMENTAL METHODS 9 Inert Atmosphere Glove Box . 9 Reagents and Solvents . • • • 9 Preparation of Compounds . • • • . • • • . 11 Preparation of Samples . • . • • • . • • 18 Computer Programs . • . • . • • . 19 Instrumentation ;, . • • • . • • • 20 III. -
Z. Chern. 1961, 1, 332-4. Vit, J. Collect. Czech. Chern. Comm
Scandium Chloride COMPONENTS: ORIGINAL MEASUREMENTS: Kirmse, E. M. (1) Scandium chloride; ScC13; [ 10361-8[:-9] ,I I Z. Chern. 1961, 1, 332-4. (2~ Uethano1; CH 40; I67-S6-1] VARIABLES: PREPARED BY: One temperature: T/K = 298.2 T. Mioduski EXPERIMENTAL VALUES: The solubility of ScC1 in methanol at 25 0 C was reported to be 3 45.5 mass % The corresponding molality calculated by the compiler is 5.52 mol kg-1 The solid phase was reported to be ScC1 .3CH 0H which could be fUrther dehydrated to SCC1 .2CH 0H (see discussion below). 3 3 3 3 COMMENTS AND/OR ADDITIONAL DATA: Since in the saturated solution there are almost 6 moles of solvent per mole of salt, the author suggests that SCC1 has a coordination number of 6. 3 AUXILIARY INFORMATION METHOD/APPARATUS/PROCEDURE: SOURCE AND PURITY OF MATERIALS: Isothermal method. About 10-15 cm3 alcohol Anhydrous ScC13 prepared by heating SC203 and activated charcoal in a stream of and ScC13 placed in glass stoppered bottles and mechanically rotated at 200 rpm in a chlorine at 900-1000oC (1). Source and thermostat for 14-15 days. After allowing purity of SC203 not specified. the equilibrated sIns to settle for 3 days, aliquots were removed for analyses. Sc Methanol was purified by fractional dis determined gravimetrically by evaporation of tillation from caS04.0.5H20. solvent followed by ignition to SC203' Identical results obtained by centrifuging the equilibrated sIns prior to analyses. Solid phase composition determined by analysis of wet residues. Samples dried in vacuum (11 ± 1 mm Hg) over P20~ at 18 ± lOC ESTIMATED ERROR: and weighed every 24 hours unt11 the mass was Soly: nothing specified. -
1. Give the Correct Names for Each of the Compounds Listed Below. A
1. Give the correct names for each of the compounds listed below. a) NaCl sodium chloride n) ZrS2 zirconium sulfide b) FrBr francium bromide o) AgI silver iodide c) KF potassium fluoride p) BaSe barium selenide d) RaS radium sulfide q) MgO magnesium oxide e) LiI lithium iodide r) LaBr3 lanthanum bromide f) Li3N lithium nitride s) Sr3N2 strontium nitride g) AlBr3 aluminum bromide t) Cd3As2 cadmium arsenide h) CdCl2 cadmium chloride u) Rb2Se rubidium selenide i) K2O potassium oxide v) Rb3N rubidium nitride j) InF3 indium fluoride w) BaF2 barium fluoride k) ZnO zinc oxide x) ZrTe2 zirconium telluride l) Y2O3 yttrium oxide y) Cs3P cesium phosphide m) CaTe calcium telluride z) Y2O3 yttrium oxide 2. Write the correct chemical formula for each of the following compounds. a) potassium bromide KBr n) potassium nitride K3N b) zinc bromide ZnBr2 o) aluminum bromide AlBr3 c) lithium iodide LiI p) zinc phosphide Zn3P2 d) scandium chloride ScCl3 q) magnesium sulfide MgS e) magnesium chloride MgCl2 r) hafnium chloride HfCl4 f) magnesium oxide MgO s) barium sulfide BaS g) hydrogen sulfide H2S t) tantalum oxide Ta2O5 h) gallium iodide GaI3 u) zirconium nitride Zr3N4 i) sodium oxide Na2O v) potassium selenide K2Se j) magnesium selenide MgSe w) germanium fluoride GeF4 k) calcium fluoride CaF2 x) francium phosphide Fr3P l) aluminum oxide Al2O3 y) zinc arsenide Zn3As2 m) beryllium chloride BeCl2 z) scandium telluride Sc2Te3 L. h. s. – Chemistry – Nomenclature – Answers – Page 1 3. Give the correct names for each of the compounds listed below. a) CaSO4 calcium -
Preparing High-Purity Anhydrous Sccl3 Molten Salt Using One-Step Rapid Heating Process
applied sciences Article Preparing High-Purity Anhydrous ScCl3 Molten Salt Using One-Step Rapid Heating Process Junhui Xiao 1,2,3,4,*, Chao Chen 1,2, Wei Ding 1, Yang Peng 1, Kai Zou 1, Tao Chen 1 and Zhiwei Zou 1 1 Sichuan Provincial Engineering Lab of Non-Metallic Mineral Powder Modification and High-Value Utilization, Southwest University of Science and Technology, Mianyang 621010, China; [email protected] (C.C.); [email protected] (W.D.); [email protected] (Y.P.); [email protected] (K.Z.); [email protected] (T.C.); [email protected] (Z.Z.) 2 Institute of Multipurpose Utilization of Mineral Resources, Chinese Academy of Geological Sciences, Chengdu 610041, China 3 Key Laboratory of Sichuan Province for Comprehensive Utilization of Vanadium and Titanium Resources, Panzhihua University, Panzhihua 617000, China 4 Key Laboratory of Ministry of Education for Solid Waste Treatment and Resource Recycle, Southwest University of Science and Technology, Mianyang 621010, China * Correspondence: [email protected]; Tel.: +86-139-9019-0544 Received: 7 July 2020; Accepted: 27 July 2020; Published: 28 July 2020 Abstract: In this study, a one-step rapid heating novel process was used to prepare high-purity anhydrous scandium chloride molten salt with low-purity scandium oxide. High-purity anhydrous ScCl3 molten salt was used as the Sc-bearing raw material for preparing the Sc-bearing master alloy. Inert gas was used to enhance the purity of anhydrous scandium chloride and reduce the hydrolysis rate of scandium. The results show that high-purity scandium chloride (purity, 99.69%) with the scandium content of 29.61%, was obtained, and the hydrolysis rate of scandium was 1.19% under the conditions used: removing ammonium chloride; residual crystal water temperature of 400 ◦C; m(Sc2O3):m(NH4Cl) = 1:2.5; holding-time of 90 min; heating-rate of 12 ◦C/min; and argon flow of 7.5 L/min. -
Periodic Table of Elements
The origin of the elements – Dr. Ille C. Gebeshuber, www.ille.com – Vienna, March 2007 The origin of the elements Univ.-Ass. Dipl.-Ing. Dr. techn. Ille C. Gebeshuber Institut für Allgemeine Physik Technische Universität Wien Wiedner Hauptstrasse 8-10/134 1040 Wien Tel. +43 1 58801 13436 FAX: +43 1 58801 13499 Internet: http://www.ille.com/ © 2007 © Photographs of the elements: Mag. Jürgen Bauer, http://www.smart-elements.com 1 The origin of the elements – Dr. Ille C. Gebeshuber, www.ille.com – Vienna, March 2007 I. The Periodic table............................................................................................................... 5 Arrangement........................................................................................................................... 5 Periodicity of chemical properties.......................................................................................... 6 Groups and periods............................................................................................................. 6 Periodic trends of groups.................................................................................................... 6 Periodic trends of periods................................................................................................... 7 Examples ................................................................................................................................ 7 Noble gases ....................................................................................................................... -
Safety Data Sheet
SAFETY DATA SHEET Revision Date 18-February-2020 Revision Number 2 1. Identification Product Name Scandium(III) chloride hexahydrate Cat No. : 11219 CAS-No 20662-14-0 Synonyms No information available Recommended Use Laboratory chemicals. Uses advised against Food, drug, pesticide or biocidal product use. Details of the supplier of the safety data sheet Company Importer/Distributor Manufacturer Fisher Scientific Alfa Aesar 112 Colonnade Road, Thermo Fisher Scientific Chemicals, Inc. Ottawa, ON K2E 7L6, 30 Bond Street, Ward Hill, MA 01835-8099 Canada Tel: 800-343-0660 Fax: 800-322-4757 Tel: 1-800-234-7437 Email: [email protected] www.alfa.com Emergency Telephone Number During normal business hours (Monday-Friday, 8am-7pm EST), call (800) 343-0660. After normal business hours, call Carechem 24 at (800) 579-7421. 2. Hazard(s) identification Classification WHMIS 2015 Classification Not classified under the Hazardous Products Regulations (SOR/2015-17) Based on available data, the classification criteria are not met Label Elements None required 3. Composition/Information on Ingredients Component CAS-No Weight % Scandium chloride (ScCl3), hexahydrate 20662-14-0 <=100 4. First-aid measures ______________________________________________________________________________________________ Page 1 / 6 Scandium(III) chloride hexahydrate Revision Date 18-February-2020 ______________________________________________________________________________________________ Eye Contact Rinse immediately with plenty of water, also under the eyelids, for at least 15 minutes. Get medical attention. Skin Contact Wash off immediately with plenty of water for at least 15 minutes. Get medical attention immediately if symptoms occur. Inhalation Remove to fresh air. Get medical attention immediately if symptoms occur. Ingestion Clean mouth with water and drink afterwards plenty of water. -
CHLORINATION and SELECTIVE VAPORIZATION of RARE EARTH ELEMENTS Daniel Gaede Montana Tech of the University of Montana
Montana Tech Library Digital Commons @ Montana Tech Graduate Theses & Non-Theses Student Scholarship Spring 2016 CHLORINATION AND SELECTIVE VAPORIZATION OF RARE EARTH ELEMENTS Daniel Gaede Montana Tech of the University of Montana Follow this and additional works at: http://digitalcommons.mtech.edu/grad_rsch Part of the Metallurgy Commons Recommended Citation Gaede, Daniel, "CHLORINATION AND SELECTIVE VAPORIZATION OF RARE EARTH ELEMENTS" (2016). Graduate Theses & Non-Theses. 72. http://digitalcommons.mtech.edu/grad_rsch/72 This Thesis is brought to you for free and open access by the Student Scholarship at Digital Commons @ Montana Tech. It has been accepted for inclusion in Graduate Theses & Non-Theses by an authorized administrator of Digital Commons @ Montana Tech. For more information, please contact [email protected]. CHLORINATION AND SELECTIVE VAPORIZATION OF RARE EARTH ELEMENTS by Daniel W. Gaede A thesis submitted in partial fulfillment of the requirements for the degree of Master of Science in Metallurgical and Materials Engineering Montana Tech 2016 i Abstract Rare earth elements are part of the lanthanide series in the periodic table. The uses and applications for these elements are ever increasing. Applications vary from smart phones to medical imaging equipment and more. Current separation techniques used today are hydrometallurgically intensive. The purpose of this study was to investigate a pyrometallurgical alternative to separate rare earth elements in ores. The proposed method, chlorination, would convert the rare earth oxides to chlorides and then apply vapor phase separation techniques to isolate the rare earth chlorides by temperature. Various reagent grade rare earth oxides were initially studied to determine chlorination efficiencies. Chlorination optimization experiments were then performed on Yb2O3, where the parameters were refined. -
Berenice González Santiago Phd Thesis
SYNTHESIS AND PROPERTIES OF SCANDIUM CARBOXYLATE METAL-ORGANIC FRAMEWORKS Berenice González Santiago A Thesis Submitted for the Degree of PhD at the University of St Andrews 2015 Full metadata for this item is available in St Andrews Research Repository at: http://research-repository.st-andrews.ac.uk/ Please use this identifier to cite or link to this item: http://hdl.handle.net/10023/6904 This item is protected by original copyright Synthesis and properties of scandium carboxylate metal-organic frameworks This thesis is submitted in partial fulfilment for the degree of Doctor of Philosophy in the Faculty of Science of the University of St Andrews by Berenice González Santiago, MSc September 2014 Supervisor: Prof. Paul A. Wright University of St Andrews Declarations 1. I, Berenice González Santiago, hereby certify that this thesis, which is approximately 40000 words in length, has been written by me, that it is the record of work carried out by me and that it has not been submitted in any previous application for a higher degree. I was admitted as a research student in September, 2010 and as a candidate for the degree of Doctor of Philosophy in October, 2011 the higher study for which this is a record was carried out in the University of St Andrews between 2010 and 2014. Date ……………………. Signature of Candidate …………………….. 2. I hereby certify that the candidate has fulfilled the conditions of the Resolution and Regulations appropriate for the degree of PhD in the University of St Andrews and that the candidate is qualified to submit this thesis in application for that degree. -
Lecture 9-12-11A
Lecture September 12 Goal for today: Chapter 3 Part II HW and Quiz due September 19th - through Section 3.6 p. 97 - naming compounds Don’t worry about hydrates Skip 3.10 for now Will NOT do Sec. 3.11 Organic Compounds at all!! CHEM131 - Fall 11 - September 12 1 Ions with Multiple Charges WebElements: the periodic table on the world-wide web http://www.webelements.com/ 1 2 3 4 5 6 7 8 9 10 11 12 13 14 15 16 17 18 hydrogen helium 1 2 H He 1.00794(7) Key: 4.002602(2) lithium beryllium element name Transition boron carbon nitrogen oxygen fluorine neon 3 4 atomic number 5 6 7 8 9 10 Li Be symbol B C N O F Ne 6.941(2) 9.012182(3) 2003 atomic weight (mean relative mass) 10.811(7) 12.0107(8) 14.0067(7) 15.9994(3) 18.9984032(5) 20.1797(6) sodium magnesium Metals aluminium silicon phosphorus sulfur chlorine argon 11 12 13 14 15 16 17 18 Na Mg Al Si P S Cl Ar 22.989770(2) 24.3050(6) 26.981538(2) 28.0855(3) 30.973761(2) 32.065(5) 35.453(2) 39.948(1) potassium calcium scandium titanium vanadium chromium manganese iron cobalt nickel copper zinc gallium germanium arsenic selenium bromine krypton 19 20 21 22 23 24 25 26 27 28 29 30 31 32 33 34 35 36 K Ca Sc Ti V Cr Mn Fe Co Ni Cu Zn Ga Ge As Se Br Kr 39.0983(1) 40.078(4) 44.955910(8) 47.867(1) 50.9415(1) 51.9961(6) 54.938049(9) 55.845(2) 58.933200(9) 58.6934(4) 63.546(3) 65.38(2) 69.723(1) 72.64(1) 74.92160(2) 78.96(3) 79.904(1) 83.798(2) rubidium strontium yttrium zirconium niobium molybdenum technetium ruthenium rhodium palladium silver cadmium indium tin antimony tellurium iodine xenon 37 38 39 40 -
Material Safety Data Sheet
LTS Research Laboratories, Inc. Safety Data Sheet Scandium (III) Chloride ––––––––––––––––––––––––––––––––––––––––––––––––––––––––––––––––––––––––––––––––––––––––––––– 1. Product and Company Identification ––––––––––––––––––––––––––––––––––––––––––––––––––––––––––––––––––––––––––––––––––––––––––––– Trade Name: Scandium (III) Chloride Chemical Formula: ScCl3 Recommended Use: Scientific research and development Manufacturer/Supplier: LTS Research Laboratories, Inc. Street: 37 Ramland Road City: Orangeburg State: New York Zip Code: 10962 Country: USA Tel #: 855-587-2436 / 855-lts-chem 24-Hour Emergency Contact: 800-424-9300 (US & Canada) +1-703-527-3887 (International) ––––––––––––––––––––––––––––––––––––––––––––––––––––––––––––––––––––––––––––––––––––––––––––– 2. Hazards Identification ––––––––––––––––––––––––––––––––––––––––––––––––––––––––––––––––––––––––––––––––––––––––––––– Signal Word: Warning Hazard Statements: H315 Causes skin irritation H319 Causes serious eye irritation H335: May cause respiratory irritation Precautionary Statements: P261: Avoid breathing dust/fume/gas/mist/vapors/spray P264: Wash face, hands, and any exposed skin thoroughly after handling. P271: Use only outdoors or in a well-ventilated area P280: Wear protective gloves/protective clothing/eye protection/face protection P302+P352: IF ON SKIN: Wash with soap and water P304+P340: IF INHALED: Remove victim to fresh air and keep at rest in a position comfortable for breathing P305+P351+P338: IF IN EYES: Rinse cautiously with water for several minutes. Remove contact -
Radiochemicals-Reagents-Catalogue
CONTENTS Company profile 4 Labeling, certificates, calibration 5 Packaging 5 Certified containers type A 6 List of radiochemicals Antimony 124Sb 7 Neodymium 147Nd 10 Arsenic 76As 7 Phosphorus 32P 11 Barium 133Ba 7 Phosphorus 32P 11 Bromine 82Br 7 Rhenium 186Re 11 Cadmium 115mCd 7 Rubidium 86Rb 11 Caesium 131Cs 7 Samarium 153Sm 11 Caesium 137Cs 8 Scandium 46Sc 11 Calcium 45Ca 8 Selenium 75Se 12 Chromium 51Cr 8 Silver 110mAg 12 Chromium 51Cr 8 Sodium 24Na 12 Chromium 51Cr EDTA 8 Strontium 85Sr 12 Cobalt 58Co 8 Strontium 89Sr 12 Cobalt 60Co 9 Strontium 90Sr 12 Copper 64Cu 9 Strontium 90Sr 13 Europium 152Eu 9 Sulphur 35S 13 Gold 198Au 9 Terbium 160Tb 13 Holmium 166Ho 9 Thallium 204TI 13 Iodine 131I 9 Thulium 170Tm 13 Indium 114mIn 10 Ytterbium 169Yb 14 Iridium 192I 10 Yttrium 90Y 14 Iron 59Fe 10 Zinc 65Zn 14 Lutetium 177Lu 10 3 Company profile Radioisotope Centre POLATOM is a part of National Centre for Nuclear Research (NCBJ). With over 60 years of experience in radioisotopes production we are focused on the following products: radiopharmaceuticals, standard solutions and reference sources, industrial sealed sources and listed here radiochemicals. At present our products are regularly used in more than 70 countries worldwide. Quality Assurance System established at the Radioisotope Centre POLATOM in the area of manufacturing, sales, dispatching and transport of radioactive materials is certified according to PN-EN ISO 9001:2015-10 Orders When ordering please refer to availability and specify at least the following information: - the specific