Functional and Morphological Evolution in Gymnosperms: a Portrait of Implicated Gene Families
Total Page:16
File Type:pdf, Size:1020Kb
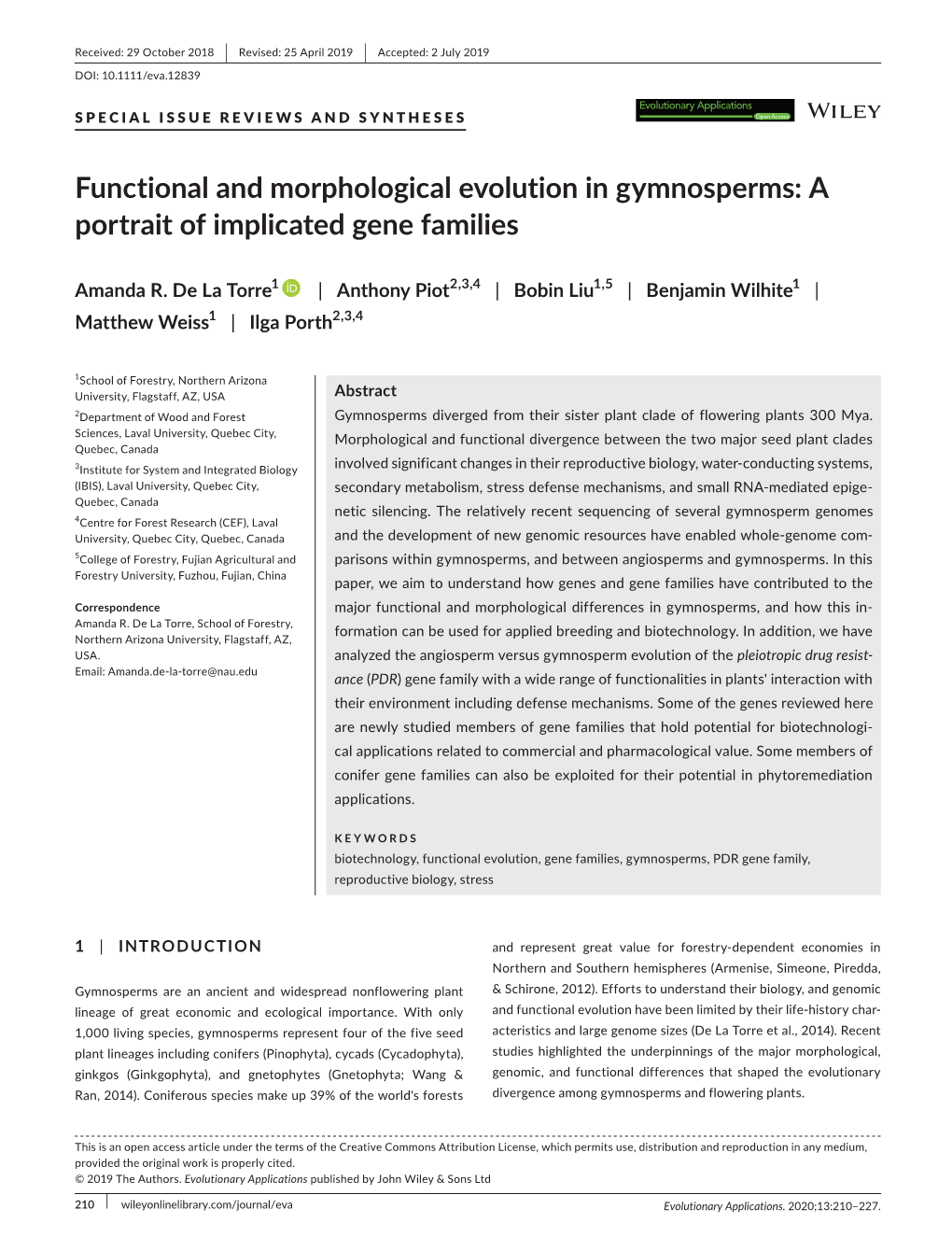
Load more
Recommended publications
-
Gymnosperms the MESOZOIC: ERA of GYMNOSPERM DOMINANCE
Chapter 24 Gymnosperms THE MESOZOIC: ERA OF GYMNOSPERM DOMINANCE THE VASCULAR SYSTEM OF GYMNOSPERMS CYCADS GINKGO CONIFERS Pinaceae Include the Pines, Firs, and Spruces Cupressaceae Include the Junipers, Cypresses, and Redwoods Taxaceae Include the Yews, but Plum Yews Belong to Cephalotaxaceae Podocarpaceae and Araucariaceae Are Largely Southern Hemisphere Conifers THE LIFE CYCLE OF PINUS, A REPRESENTATIVE GYMNOSPERM Pollen and Ovules Are Produced in Different Kinds of Structures Pollination Replaces the Need for Free Water Fertilization Leads to Seed Formation GNETOPHYTES GYMNOSPERMS: SEEDS, POLLEN, AND WOOD THE ECOLOGICAL AND ECONOMIC IMPORTANCE OF GYMNOSPERMS The Origin of Seeds, Pollen, and Wood Seeds and Pollen Are Key Reproductive SUMMARY Innovations for Life on Land Seed Plants Have Distinctive Vegetative PLANTS, PEOPLE, AND THE Features ENVIRONMENT: The California Coast Relationships among Gymnosperms Redwood Forest 1 KEY CONCEPTS 1. The evolution of seeds, pollen, and wood freed plants from the need for water during reproduction, allowed for more effective dispersal of sperm, increased parental investment in the next generation and allowed for greater size and strength. 2. Seed plants originated in the Devonian period from a group called the progymnosperms, which possessed wood and heterospory, but reproduced by releasing spores. Currently, five lineages of seed plants survive--the flowering plants plus four groups of gymnosperms: cycads, Ginkgo, conifers, and gnetophytes. Conifers are the best known and most economically important group, including pines, firs, spruces, hemlocks, redwoods, cedars, cypress, yews, and several Southern Hemisphere genera. 3. The pine life cycle is heterosporous. Pollen strobili are small and seasonal. Each sporophyll has two microsporangia, in which microspores are formed and divide into immature male gametophytes while still retained in the microsporangia. -
Plant Classification
Plant Classification Vascular plants are a group that has a system Non-Vascular plants are low growing plants of tubes (roots, stems and leaves) to help that get materials directly from their them transport materials throughout the surroundings. They have small root-like plant. Tubes called xylem move water from structures called rhizoids which help them the roots to the stems and leaves. Tubes adhere to their substrate. They undergo called phloem move food from the leaves asexual reproduction through vegetative (where sugar is made during propagation and sexual reproduction using photosynthesis) to the rest of the plant’s spores. Examples include bryophytes like cells. Vascular plants reproduce asexually hornworts, liverworts, and mosses. through spores and vegetative propagation (small part of the plant breaks off and forms a new plant) and sexually through pollen (sperm) and ovules (eggs). A gymnosperm is a vascular plant whose An angiosperm is a vascular plant whose seeds are not enclosed in an ovule or fruit. mature seeds are enclosed in a fruit or The name means “naked seed” and the ovule. They are flowering plants that group typically refers to conifers that bear reproduce using seeds and are either male and female cones, have needle-like “perfect” and contain both male and female leaves and are evergreen (leaves stay green reproductive structures or “imperfect” and year round and do not drop their leaves contain only male or female structures. during the fall and winter. Examples include Angiosperm trees are also called hardwoods pine trees, ginkgos and cycads. and they have broad leaves that change color and drop during the fall and winter. -
B.Sc Botany (Sub.) I Group: a General Characters of Gymnosperm
1 B.Sc Botany (Sub.) I Group: A General Characters of Gymnosperm Gymnosperms are a small group of plants comprising only 70 genera and 725 living species. The word Gymnosperm was used by the Greek botanists Theophrastus in 300 B.C. for the plants with unprotected seeds. Gymnosperms are naked seeded plants. The gymnosperms are characterized by the following features: i. It shows the distinct alternation of generations. ii. Sporophytic generation is the dormant phase of the life cycle. The main plants are sporophyte and the gametophytes are dependent on it throughout. iii. The sporophytic plants are usually tall, woody, perennial trees or shrubs and differentiated into root, stem and leaves. iv. Root- Root is usually well developed tap root system. The stele in root is diarch or polyarch. v. Stem- stems are usually branched but unbranched in Cycas. vi. The leaves may be compound as in Cycas or simple as in Pinus. Internal structure: i. The vascular bundles in stem are arranged in a ring. The bundles are conjoint, collateral and open. ii. The secondary growth is effected by a cambial layer which produces secondary xylem and secondary phloem on the inner and outer side respectively. iii. The secondary wood forming distinct annual ring. Spring wood: It is made up of layer tracheids with the walls and layer lumen. Autumn wood: It is made up of compactly arranged smaller tracheids with thick walls and smaller lumen. iv. The wood may be manoxylic or pycnoxylic and may be monoxylic or polyxylic. Dr. Sanjeev Kumar Vidyarthi, dept. of Botany, Dr. L.K.V.D. -
A Global Analysis of the Distribution and Conservation Status Of
Journal of Biogeography (J. Biogeogr.) (2015) 42, 809–820 SYNTHESIS Fighting their last stand? A global analysis of the distribution and conservation status of gymnosperms Yann Fragniere1,Sebastien Betrisey2,3,Leonard Cardinaux1, Markus Stoffel4,5 and Gregor Kozlowski1,2* 1Natural History Museum Fribourg, CH-1700 ABSTRACT Fribourg, Switzerland, 2Department of Biology Aim Gymnosperms are often described as a marginal and threatened group, and Botanic Garden, University of Fribourg, members of which tend to be out-competed by angiosperms and which therefore CH-1700 Fribourg, Switzerland, 3Conservation Biogeography, Department of preferentially persist at higher latitudes and elevations. The aim of our synthesis Geosciences, University of Fribourg, CH-1700 was to test these statements by investigating the global latitudinal and elevational Fribourg, Switzerland, 4Dendrolab.ch, distribution of gymnosperms, as well as their conservation status, using all extant Institute of Geological Sciences, University of gymnosperm groups (cycads, gnetophytes, ginkgophytes and conifers). 5 Bern, CH-3012 Bern, Switzerland, Institute Location Worldwide. for Environmental Sciences, Climatic Change and Climate Impacts, University of Geneva, Methods We developed a database of 1014 species of gymnosperms containing CH-1227 Carouge, Switzerland latitudinal and elevational distribution data, as well as their global conservation status, as described in the literature. The 1014 species comprised 305 cycads, 101 gnetophytes, the only living representative of ginkgophytes, and 607 conifers. Generalized additive models, frequency histograms, kernel density estimations and distribution maps based on Takhtajan’s floristic regions were used. Results Although the diversity of gymnosperms decreases at equatorial lati- tudes, approximately 50% of the extant species occur primarily between the tropics. More than 43% of gymnosperms can occur at very low elevations (≤ 200 m a.s.l.). -
Name That Gymnosperm
A B C D This tree is found frequently This evergreen is found throughout This species is found at drier and This Wyoming tree is a remnant of throughout the eastern half of the state. The species is known for lower elevations compared to some the last ice age and is found exclu- Wyoming ranging from the Black often having serotinous cones that of the other trees pictured. This tree sively in the Black Hills of Wyoming Hills to the Laramie Mountains and only open to release seeds when the shares its name with the town in and South Dakota. It grows at higher the Bighorns. The tree is known cone is heated. Once the limbs are central Wyoming. elevations along riparian or wet areas for its ability to withstand the heat removed, this tree makes an excel- in its native habitat. of wildfires because of its thick, lent pole for teepees because of its reddish-colored bark. long, slender trunk. NAME THAT GYMNOSPERM Wyoming is host to many conifer (gymnosperm or “naked in hot, dry, and low elevations while others are found in cold and seed” plants including conifer, cycads, and ginkos) tree species. high elevations. Cones are an excellent tool that can be used for The cones in this quiz and their parent trees are found at different identification of evergreens. Match the tree to the photo. Good geographical locations across the Cowboy State. Some are found luck and keep an eye out for these trees this year! E F G H Known for having extremely flexible This species is probably better known This tree is found in most high- This tree grows at high elevations limbs, this tree is often found brav- as an ornamental in Wyoming but is elevation forests of Wyoming. -
Workshop on Alternation of Generations by Dana Krempels
Workshop on Alternation of Generations by Dana Krempels Introduction For students new to the study of Plantae, the life cycle of plants--in which a diploid generation alternates with a haploid generation--can be difficult to understand. In this workshop, you will (1) examine the details of plant gametophyte and sporophyte structure and function, and (2) create an animal analog to this type of life history. Your goals: 1. Understand the alternation of haploid and diploid individuals in the plant life cycle. 2. Understand the terminology used to describe parts of the life cycle, and recognize what each life cycle stage looks like in the major plant taxa. 3. Acquire a more "personal" understanding of how the alternation of generations works by designing an imaginary animal that goes through this type of life cycle. I. Alternation of Generations in Plants: Processes and Terminology The painful part comes first: knowing the general course of events, and what each life cycle stage and structure is called. A. An Overview of Alternation of Generations In the plant life cycle, generations alternate between a diploid (2n) sporophyte and a haploid (n) gametophyte. Essentially, the sporophyte bears gametophyte offspring, and the gametophyte bears sporophyte offspring. The plants alternate ploidy from hapoid to diploid in each generation. The sporophyte and gametophye generations look completely different from one another, as you can see from the diagram below. 1. In the diagram below, use the following terms to label the organisms/structures marked a – e. gametophyte spore zygote sporophyte gamete 2. In the spaces labeled 1 – 5, insert the appropriate term, choosing from these three: meiosis mitosis fertilization 3. -
Protista and Fungi
Biology 2 – Practicum 2 1 Biology 2 Lab Packet For Practical 2 Biology 2 – Practicum 2 2 PLANT CLASSIFICATION: Domain: Eukarya (Supergroup: Archaeplastida) Kingdom: Plantae Nonvascular Seedless Plants Division: Hepatophyta - Liverworts Division: Bryophyta - Mosses Vascular Seedless Plants Division: Lycophyta - Club Mosses Division: Pterophyta (Psilophyta) - Whisk ferns (Sphenophyta) - Horsetails (Pterophyta) - Ferns Red Algae Archaeplastida Chlorophytes Charophyceans Liverworts Plants Mosses Hornworts Lycophytes Pterophytes Gymnosperms INTRODUCTION TO PLANTS Angiosperms The kingdom Plantae includes about twelve divisions. They are placed in the clade Archaeplastida along with the green algae and charophytes. They are all eukaryotic and multicellular with distinct cell walls. Photosynthetic pigments occur in organelles called plastids. Plants have adapted to the terrestrial environment with an increase in structural complexity. Many plants have developed organs for anchorage, conduction, support, and photosynthesis. Reproduction is primarily sexual, with an alternation of generation of haploid and diploid generations. The sporophyte generation becomes increasingly predominant as plants evolve. The nonvascular plants lack conductive tissue and are limited to a specific range of terrestrial habitats. These plants display two adaptations that first made the move onto land possible. They possess a waxy cuticle to reduce water loss and their gametes develop within gametangia for protection of the embryo. These plants are limited in range because they require water for reproduction. They lack vascular tissue, which means they must live in moist environments and lack woody structures for support therefore they grow low to the ground. Station 1 – Kingdom Planate 1. What supergroup do they belong to and what characteristic are responsible for this positioning? 2. What characteristics are specific to plants? 3. -
Reproductive Morphology
Week 3; Wednesday Announcements: 1st lab quiz TODAY Reproductive Morphology Reproductive morphology - any portion of a plant that is involved with or a direct product of sexual reproduction Example: cones, flowers, fruits, seeds, etc. Basic Plant Life cycle Our view of the importance of gametes in the life cycle is shaped by the animal life cycle in which meiosis (the cell division creating haploid daughter cells with only one set of chromosomes) gives rise directly to sperm and eggs which are one celled and do not live independently. Fertilization (or the fusion of gametes – sperm and egg) occurs inside the animal to recreate the diploid organism (2 sets of chromosomes). Therefore, this life cycle is dominated by the diploid generation. This is NOT necessarily the case among plants! Generalized life cycle -overhead- - alternation of generations – In plants, spores are the result of meiosis. These may grow into a multicellular, independent organism (gametophyte – “gamete-bearer”), which eventually produces sperm and eggs (gametes). These fuse (fertilization) and a zygote is formed which grows into what is known as a sporophyte - “spore-bearer”. (In seed plants, pollination must occur before fertilization! ) This sporophyte produces structures called sporangia in which meiosis occurs and the spores are released. Spores (the product of meiosis) are the first cell of the gametophyte generation. Distinguish Pollination from Fertilization and Spore from Gamete Pollination – the act of transferring pollen from anther or male cone to stigma or female cone; restricted to seed plants. Fertilization – the act of fusion between sperm and egg – must follow pollination in seed plants; fertilization occurs in all sexually reproducing organisms. -
1 Relationships of Angiosperms To
Relationships of Angiosperms to 1 Other Seed Plants Seed plants are of fundamental importance both evolution- all gymnosperms (living and extinct) together are not arily and ecologically. They dominate terrestrial landscapes, monophyletic. Importantly, several fossil lineages, Cayto- and the seed has played a central role in agriculture and hu- niales, Bennettitales, Pentoxylales, and Glossopteridales man history. There are fi ve extant lineages of seed plants: (glossopterids), have been proposed as putative close rela- angiosperms, cycads, conifers, gnetophytes, and Ginkgo. tives of the angiosperms based on phylogenetic analyses These fi ve groups have usually been treated as distinct (e.g., Crane 1985; Rothwell and Serbet 1994; reviewed in phyla — Magnoliophyta (or Anthophyta), Cycadophyta, Doyle 2006, 2008, 2012; Friis et al. 2011). These fossil lin- Co ni fe ro phyta, Gnetophyta, and Ginkgophyta, respec- eages, sometimes referred to as the para-angiophytes, will tively. Cantino et al. (2007) used the following “rank- free” therefore be covered in more detail later in this chapter. An- names (see Chapter 12): Angiospermae, Cycadophyta, other fossil lineage, the corystosperms, has been proposed Coniferae, Gnetophyta, and Ginkgo. Of these, the angio- as a possible angiosperm ancestor as part of the “mostly sperms are by far the most diverse, with ~14,000 genera male hypothesis” (Frohlich and Parker 2000), but as re- and perhaps as many as 350,000 (The Plant List 2010) to viewed here, corystosperms usually do not appear as close 400,000 (Govaerts 2001) species. The conifers, with ap- angiosperm relatives in phylogenetic trees. proximately 70 genera and nearly 600 species, are the sec- The seed plants represent an ancient radiation, with ond largest group of living seed plants. -
Biol 211 (2) Chapter 31 October 9Th Lecture
S.I. Biol 211 Biology 211 (2) Week 7! Chapter 31! ! VOCABULARY! Practice: http://www.superteachertools.us/speedmatch/speedmatch.php? gamefile=4106#.VhqUYGRVhBc ! Alternation of Angiosperm: A Antheridia: The Archegonia: The generations: A life cycle flowering vascular sperm producing egg-producing involving alternation of a plant that produces structure in most structure in most multicellular haploid seed within mature land plants except land plants except ovaries (fruits). The angiosperms angiosperms stage (gametophyte) with angiosperms form a a multicellular diploid single lineage stage (sporophyte). Occurs in most plants and some protists. Artificial selection: Bisexual Carpel: The female Diploid: Having two Deliberate manipulation gametophyte: One reproductive organ sets of by humans, as in animal gametophyte that in a flower, chromosomes (2n) and plant breeding, of produces both eggs contains the ovary, the genetic composition and sperm which contains of a population by ovules, which allowing only individuals contain the with desirable traits to megasporangia reproduce Double fertilization: An Endosperm: A Fruit: In Gametangia: The unusual form of triploid (3n) tissue angiosperms, a gamete-forming reproduction seen in in the seed of a mature, ripened structure found in flowering plants, in which flowering plant plant ovary, along all land plants one sperm cell fuses with an (angiosperm) that with the seeds it except angiosperms. egg to form a zygote and the serves as food for contains and Contains an other sperm cell fuses with two polar nuclei to form the the plant embryo. adjacent fused antheridium and triploid endosperm Functionally parts, often archegonium. analogous to the functions in seed yolk of an egg dispersal Gametophyte: In Gymnosperm: A Haploid: Having Heterospory: In organisms undergoing vascular plant that one set of seed plants, the alternation of makes seeds but chromosomes production of two generations, the does not produce distinct types of multicellular haploid form flowers. -
Diversity of Plants Monophyly
Dr. Mitch Pavao-Zuckerman Department of Ecology and Evolutionary Biology Diversity of Plants 621-8220 [email protected] Office hours: Biosciences West 431 W and F 1-2 p.m. or by appointment Diversity of Plants (Fig 29.4) Monophyly Chlorophyta Ancestral Alga • Monophyletic group –includes the most recent common ancestor and all Nontracheophytes decendents • These are NOT monophyletic: Nonseed Tracheophytes Gymnosperms The Transition to Life on Land Angiosperms The Vascular Plants The Seed Plants The Flowering Plants Green Plants Embryophytes (Land Plants) (viridiphytes) are a monophyletic group Land Plants are also a monophyletic group • Photosynthetic eukaryotes that use • Green Plants include the chlorophyll a and b and store Chlorophytes (green algae) carbohydrates starch • Other green algae • and the land plants •Resting embryo with placental connection to the parent. 1 The Conquest of the Land The Conquest of the Land History of plants on land Early innovations in land plant • 500 mya - a few algae and lichens. evolution: • By 460 mya - primitive Land Plants, 1. cuticle (waxy coating) • By 425 mya - Early Vascular Plants were common 2. thick spore wall • How did it happen? 3. Antheridia and archegonia •Obstacles? Reconstruction (gamete cases), 4. protected embryo Fossil 5. protective pigments – flavonoids absorb damaging UV light Land Plants (Embryophytes) (Fig 29.4) Nontracheophytes: Chlorophyta Ancestral Alga Liverworts, Hornworts, and Mosses Nontracheophytes • Small plants (compared to present day Protected shrubs and trees) Embryos • Lack specialized water (xylem) and food Nonseed Tracheophytes conducting tubes (phloem) of vascular plants. Gymnosperms •Rely on diffusion of water and minerals. Angiosperms Plant Kingdom? Plant life cycles feature alternation of Nontracheophytes: generations (Fig 29.2) Liverworts, Hornworts, and Mosses Multicellular Haploid • Diploid generation is Fig. -
Phylogenetic Relationship and Domain Organisation of SET Domain Proteins of Archaeplastida Supriya Sarma* and Mukesh Lodha*
Sarma and Lodha BMC Plant Biology (2017) 17:238 DOI 10.1186/s12870-017-1177-1 RESEARCHARTICLE Open Access Phylogenetic relationship and domain organisation of SET domain proteins of Archaeplastida Supriya Sarma* and Mukesh Lodha* Abstract Background: SET is a conserved protein domain with methyltransferase activity. Several genome and transcriptome data in plant lineage (Archaeplastida) are available but status of SET domain proteins in most of the plant lineage is not comprehensively analysed. Results: In this study phylogeny and domain organisation of 506 computationally identified SET domain proteins from 16 members of plant lineage (Archaeplastida) are presented. SET domain proteins of rice and Arabidopsis are used as references. This analysis revealed conserved as well as unique features of SET domain proteins in Archaeplastida. SET domain proteins of plant lineage can be categorised into five classes- E(z), Ash, Trx, Su(var) and Orphan. Orphan class of SET proteins contain unique domains predominantly in early Archaeplastida. Contrary to previous study, this study shows first appearance of several domains like SRA on SET domain proteins in chlorophyta instead of bryophyta. Conclusion: The present study is a framework to experimentally characterize SET domain proteins in plant lineage. Keywords: Archaeplastida, Histone modifications, Epigenetics, SET domain, Polycomb, Phylogenetic analysis, Evolution Background deposited by DOT1 methyltransferase that does not Epigenetic cellular memory is heritable during mitosis and/ contain SET domain [13]. meiosis but is not encoded in the genetic material [1, 2]. Based on SET domain sequence similarity to Drosoph- Histone post-translational modifications, DNA methyla- ila homologues, plant SET domain proteins are classified tion, and non-coding RNAs and chromatin remodelling into 4 main classes [14], Enhancer of Zeste, E(z) homo- are the major components of epigenetic inheritance [3].