Melatonin Synthesis and Signaling for Seasonal Reproductive Timing
Total Page:16
File Type:pdf, Size:1020Kb
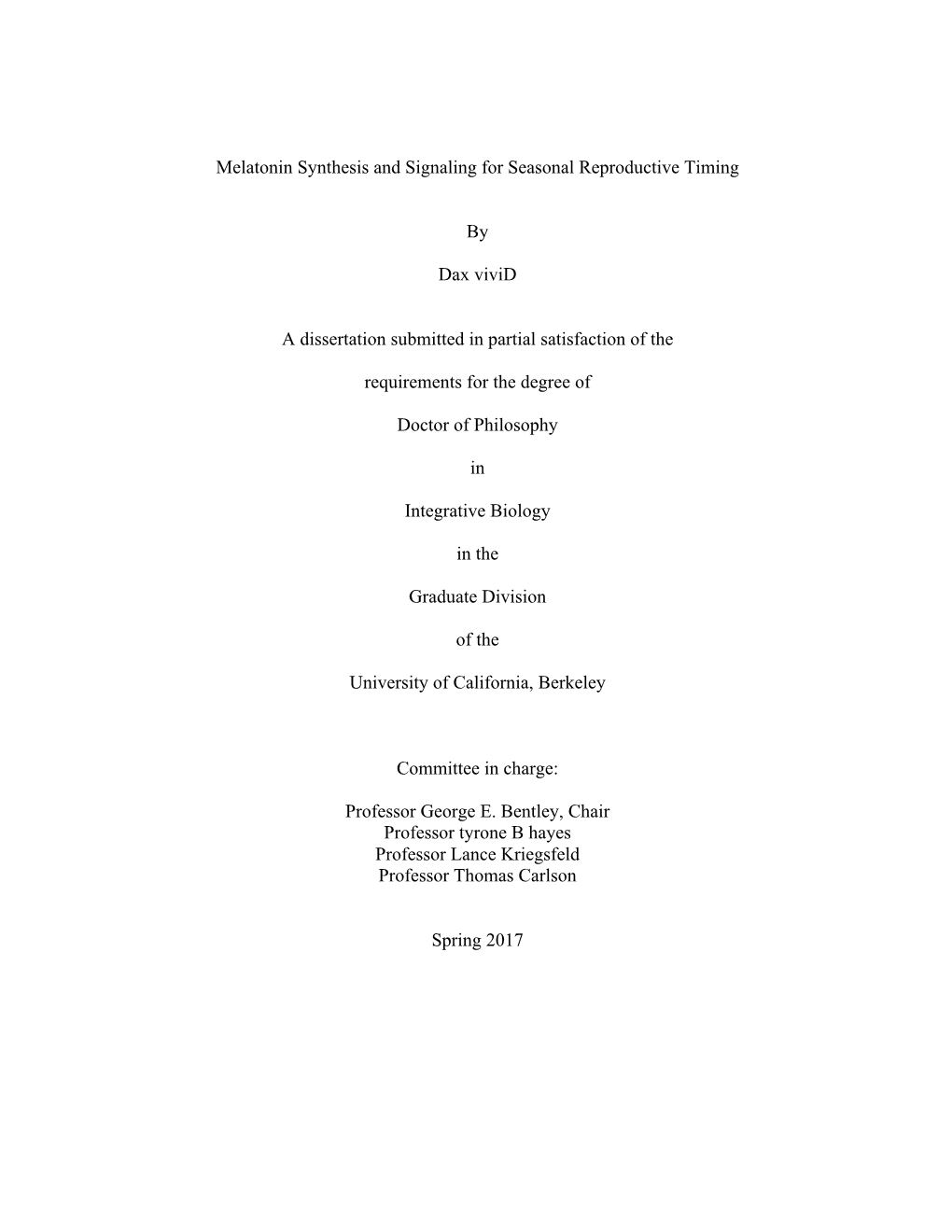
Load more
Recommended publications
-
Reproductive Seasonality in Captive Wild Ruminants: Implications for Biogeographical Adaptation, Photoperiodic Control, and Life History
Zurich Open Repository and Archive University of Zurich Main Library Strickhofstrasse 39 CH-8057 Zurich www.zora.uzh.ch Year: 2012 Reproductive seasonality in captive wild ruminants: implications for biogeographical adaptation, photoperiodic control, and life history Zerbe, Philipp Abstract: Zur quantitativen Beschreibung der Reproduktionsmuster wurden Daten von 110 Wildwiederkäuer- arten aus Zoos der gemässigten Zone verwendet (dabei wurde die Anzahl Tage, an denen 80% aller Geburten stattfanden, als Geburtenpeak-Breite [BPB] definiert). Diese Muster wurden mit verschiede- nen biologischen Charakteristika verknüpft und mit denen von freilebenden Tieren verglichen. Der Bre- itengrad des natürlichen Verbreitungsgebietes korreliert stark mit dem in Menschenobhut beobachteten BPB. Nur 11% der Spezies wechselten ihr reproduktives Muster zwischen Wildnis und Gefangenschaft, wobei für saisonale Spezies die errechnete Tageslichtlänge zum Zeitpunkt der Konzeption für freilebende und in Menschenobhut gehaltene Populationen gleich war. Reproduktive Saisonalität erklärt zusätzliche Varianzen im Verhältnis von Körpergewicht und Tragzeit, wobei saisonalere Spezies für ihr Körpergewicht eine kürzere Tragzeit aufweisen. Rückschliessend ist festzuhalten, dass Photoperiodik, speziell die abso- lute Tageslichtlänge, genetisch fixierter Auslöser für die Fortpflanzung ist, und dass die Plastizität der Tragzeit unterstützend auf die erfolgreiche Verbreitung der Wiederkäuer in höheren Breitengraden wirkte. A dataset on 110 wild ruminant species kept in captivity in temperate-zone zoos was used to describe their reproductive patterns quantitatively (determining the birth peak breadth BPB as the number of days in which 80% of all births occur); then this pattern was linked to various biological characteristics, and compared with free-ranging animals. Globally, latitude of natural origin highly correlates with BPB observed in captivity, with species being more seasonal originating from higher latitudes. -
Estrous Cycle in Equine and Camels
Prepared by- Dr. S. K. Sheetal Assistant Professor cum Jr. Scientist Department of Veterinary Gynaecology and Obstetrics, Bihar Veterinary College, Bihar Animal Sciences University, Patna-800014 1 Estrous Cycle of Mare 2 Terminology "Estrous" or Ostrous refers to the entire cycle. "Estrus" or Oestrus refers to the "heat" stage of that cycle when the mare is receptive to the stallion "Diestrus" (diœstrus) Period in between the estrus phases when the mare is not receptive to the stallion "Anestrus" (anœstrus) Compete absence of estrus. Long Day Breeder The mare is a "seasonally polyestrus" animal undergoes regular estrus cycles during a portion of the year (late spring, summer and early fall) Not in winter. This is nature's way of preventing the arrival of a foal during bad weather. 3 Signs of Estrus • Vulva become large and swollen, scarlet or orange colour, wet, glossy, covered with mucous. • Labial folds Loose • Vaginal mucosa highly vascular • Tail raised. • Urine expelled in small amount frequently • Clitoris is exposed by prolonged rhythmic contraction Known as Winking of clitoris • Ovulatory Cycle 21 days • Luteal phase= 14 days • Follicular or estrous phase = 7 days 4 Long Duration Estrus in Mare It may be due to Ovary surrounded by serous coat and takes longer time for migration of follicles to reach the ovulation fossa to rupture. Preovulatory follicle requires longer time to reach maximal size (due to less sensitiveness of ovary with exogenous FSH) Low level of LH delays ovulation 5 Ovulation • Most ovulations days 3, 4,or 5 of estrus, 24 to 48 hours before the end of behavioral estrus • Time of ovulation is more closely related to the end than to the onset of estrus. -
Chapter One: Introduction
Nocturnal Adventures Curriculum Manual 2013 Updated by Kimberly Mosgrove 3/28/2013 1 TABLE OF CONTENTS CHAPTER 1: INTRODUCTION……………………………………….……….…………………… pp. 3-4 CHAPTER 2: THE NUTS AND BOLTS………………………………………….……………….pp. 5-10 CHAPTER 3: POLICIES…………………………………………………………………………………….p. 11 CHAPTER 4: EMERGENCY PROCEDURES……………..……………………….………….pp. 12-13 CHAPTER 5: GENERAL PROGRAM INFORMATION………………………….………..pp.14-17 CHAPTER 6: OVERNIGHT TOURS I - Animal Adaptations………………………….pp. 18-50 CHAPTER 7: OVERNIGHT TOURS II - Sleep with the Manatees………..………pp. 51-81 CHAPTER 8: OVERNIGHT TOURS III - Wolf Woods…………….………….….….pp. 82-127 CHAPTER 9: MORNING TOURS…………………………………………………………….pp.128-130 Updated by Kimberly Mosgrove 3/28/2013 2 CHAPTER ONE: INTRODUCTION What is the Nocturnal Adventures program? The Cincinnati Zoo and Botanical Garden’s Education Department offers a unique look at our zoo—the zoo at night. We offer three sequential overnight programs designed to build upon students’ understanding of the natural world. Within these programs, we strive to combine learning with curiosity, passion with dedication, and advocacy with perspective. By sharing our knowledge of, and excitement about, environmental education, we hope to create quality experiences that foster a sense of wonder, share knowledge, and advocate active involvement with wildlife and wild places. Overnight experiences offer a deeper and more profound look at what a zoo really is. The children involved have time to process what they experience, while encountering firsthand the wonderful relationships people can have with wild animals and wild places. The program offers three special adventures: Animal Adaptations, Wolf Woods, and Sleep with the Manatees, including several specialty programs. Activities range from a guided tour of zoo buildings and grounds (including a peek behind-the-scenes), to educational games, animal demonstrations, late night hikes, and presentations of bio-facts. -
Accounting for Intraspecific Variation Transforms Our Understanding of Artiodactyl Social Evolution
ACCOUNTING FOR INTRASPECIFIC VARIATION TRANSFORMS OUR UNDERSTANDING OF ARTIODACTYL SOCIAL EVOLUTION By Monica Irene Miles Loren D. Hayes Hope Klug Associate Professor of UC Foundation Associate Professor of Biology, Geology, and Environmental Science Biology, Geology, and Environmental Science (Chair) (Committee Member) Timothy Gaudin UC Foundation Professor of Biology, Geology, and Environmental Science (Committee Member) ACCOUNTING FOR INTRASPECIFIC VARIATION TRANSFORMS OUR UNDERSTANDING OF ARTIODACTYL SOCIAL EVOLUTION By Monica Irene Miles A Thesis Submitted to the Faculty of the University of Tennessee at Chattanooga in Partial Fulfillment of the Requirements of the Degree of Master of Science: Environmental Science The University of Tennessee at Chattanooga Chattanooga, Tennessee December 2018 ii Copyright © 2018 By Monica Irene Miles All Rights Reserved iii ABSTRACT A major goal in the study of mammalian social systems has been to explain evolutionary transitions in social traits. Recent comparative analyses have used phylogenetic reconstructions to determine the evolution of social traits but have omitted intraspecific variation in social organization (IVSO) and mating systems (IVMS). This study was designed to summarize the extent of IVSO and IVMS in Artiodactyla and Perissodactyla, and determine the ancestral social organization and mating system for Artiodactyla. Some 82% of artiodactyls showed IVSO, whereas 31% exhibited IVMS; 80% of perissodactyls had variable social organization and only one species showed IVMS. The ancestral population of Artiodactyla was predicted to have variable social organization (84%), rather than solitary or group-living. A clear ancestral mating system for Artiodactyla, however, could not be resolved. These results show that intraspecific variation is common in artiodactyls and perissodactyls, and suggest a variable ancestral social organization for Artiodactyla. -
Girard-Buttoz Et Al. 2009.Pdf
Physiology & Behavior 98 (2009) 168–175 Contents lists available at ScienceDirect Physiology & Behavior journal homepage: www.elsevier.com/locate/phb Seasonal and social influences on fecal androgen and glucocorticoid excretion in wild male long-tailed macaques (Macaca fascicularis) C. Girard-Buttoz a, M. Heistermann a, S. Krummel a,b, A. Engelhardt a,c,⁎ a Department of Reproductive Biology, German Primate Centre, 37077 Göttingen, Germany b Faculty of Biology, Georg-August-University Göttingen, 37073 Göttingen, Germany c Institute for Human Biology and Anthropology, Free University of Berlin, 14195 Berlin, Germany article info abstract Article history: Whereas it is well known that in strictly seasonal breeding primates (income breeders), alike other Received 28 November 2008 vertebrates, males show pronounced changes in testicular and adrenal hormone levels concurrent with Received in revised form 7 May 2009 reproductive activity, hormonal patterns in males of non-strictly seasonal breeding primate species (capital Accepted 13 May 2009 breeders) and their relation to seasonal and social correlates remain largely unknown. In the present study, we examined the annual pattern of fecal androgen and glucocorticoid excretion and their relationship to Keywords: environmental (rainfall, temperature) and social factors (number of cycling females, male aggression and Long-tailed macaques Macaca fascicularis copulation rates, male dominance rank) in a group of wild long-tailed macaques (Macaca fascicularis), a Glucocorticoids species with a moderate degree of reproductive seasonality and classified as capital breeder. The study was Androgens carried out in the Gunung Leuser National Park, North Sumatra, Indonesia over a period of ten months Seasonality encompassing the conception and the birth season. -
Redalyc.CAMELS' REPRODUCTIVE and PHYSIOLOGICAL PERFORMANCE TRAITS AS AFFECTED by ENVIRONMENTAL CONDITIONS
Tropical and Subtropical Agroecosystems E-ISSN: 1870-0462 [email protected] Universidad Autónoma de Yucatán México Marai, I.F.M.; Zeidan, A.E.B.; Abdel-Samee, A.M.; Abizaid, A.; Fadiel, A. CAMELS' REPRODUCTIVE AND PHYSIOLOGICAL PERFORMANCE TRAITS AS AFFECTED BY ENVIRONMENTAL CONDITIONS Tropical and Subtropical Agroecosystems, vol. 10, núm. 2, mayo-agosto, 2009, pp. 129-149 Universidad Autónoma de Yucatán Mérida, Yucatán, México Available in: http://www.redalyc.org/articulo.oa?id=93912989002 How to cite Complete issue Scientific Information System More information about this article Network of Scientific Journals from Latin America, the Caribbean, Spain and Portugal Journal's homepage in redalyc.org Non-profit academic project, developed under the open access initiative Tropical and Subtropical Agroecosystems, 10 (2009): 129 - 149 REVIEW [REVISIÓN] CAMELS’ REPRODUCTIVE AND PHYSIOLOGICAL PERFORMANCE Tropical and TRAITS AS AFFECTED BY ENVIRONMENTAL CONDITIONS [EFECTO DE LAS CONDICIONES AMBIENTALES SOBRE Subtropical CARACTERÍSTICAS REPRODUCTIVAS Y FISIOLÓGICAS DEL CAMELLO] Agroecosystems I.F.M. Marai1*, A.E.B. Zeidan2, A.M. Abdel-Samee3, A. Abizaid4 and A. Fadiel4 1Department of Animal Production, Faculty of Agriculture, Zagazig University, Zagazig, Egypt. 2 Animal Production Research Institute, Dokki, Giza, Egypt. 3.Department of Animal Production, Faculty of Environmental Agricultural Sciences, Suez Canal University, El-Arish, Egypt. 4Yale University, School of Medcicine, OBGYN Dept., and Center for Reproductive Biology, New Haven, CT., 06511, USA *Corresponding author SUMMARY importance of SCN in reproductive functions of the camel, are needed. The male camel is described as a seasonal breeder with a marked peak in sexual activity (the rut) during the Key words: breeding and non-breeding seasons, breeding season and it is generally thought that the Dromedary camel, males and females, physiological male is sexually quiescent for the remainder of the background, reproductive activity. -
Understanding Mare Reproduction
Know how. Know now. EC271 (Revised October 2011) UNDERSTANDING MARE REPRODUCTION Kathy Anderson Extension Horse Specialist University of Nebraska–Lincoln Extension is a Division of the Institute of Agriculture and Natural Resources at the University of Nebraska–Lincoln cooperating with the Counties and the United States Department of Agriculture. University of Nebraska–Lincoln Extension educational programs abide with the nondiscrimination policies of the University of Nebraska–Lincoln and the United States Department of Agriculture. © 1994-2011, The Board of Regents of the University of Nebraska on behalf of the University of Nebraska–Lincoln Extension. All rights reserved. UNDERSTANDING MARE REPRODUCTION Kathy Anderson Extension Horse Specialist University of Nebraska–Lincoln INTRODUCTION FUNCTIONAL ANATOMY Many producers who raise horses find breeding A correctly functioning reproductive tract is es- mares rewarding, yet frustrating. Mares and stal- sential to the potential fertility of a broodmare. The lions are traditionally placed in the breeding herd tract goes through various changes as a mare exhib- due to successful performance records, with little its estrous cycles. A good working knowledge of a consideration for their reproductive capabilities. mare’s anatomy and these changes will aid in early Horses are difficult breeders with an estimated identification of potential abnormalities. These foaling rate of below 60 percent. Various factors changes can easily be monitored through rectal pal- contribute to this, including long-erratic estrous pation or ultrasound by a veterinarian. cycles and an imposed breeding season that does The rectum is located above the reproductive not coincide with the mare’s natural breeding sea- tract allowing for a noninvasive examination of the son. -
Effects of Melatonin on Anterior Pituitary Plasticity: a Comparison Between Mammals and Teleosts
REVIEW published: 11 January 2021 doi: 10.3389/fendo.2020.605111 Effects of Melatonin on Anterior Pituitary Plasticity: A Comparison Between Mammals and Teleosts Elia Ciani 1, Trude M. Haug 2, Gersende Maugars 3, Finn-Arne Weltzien 3, Jack Falco´ n 4 and Romain Fontaine 3* 1 Department of Pharmacy, Faculty of Mathematics and Natural Sciences, University of Oslo, Oslo, Norway, 2 Department of Oral Biology, Faculty of Dentistry, University of Oslo, Oslo, Norway, 3 Physiology Unit, Faculty of Veterinary Medicine, Edited by: Norwegian University of Life Sciences, Oslo, Norway, 4 Laboratoire Biologie des Organismes et Ecosystèmes Aquatiques Vance L. Trudeau, (BOREA), MNHN, CNRS FRE 2030, SU, IRD 207, UCN, UA, Paris, France University of Ottawa, Canada Reviewed by: He´ lène Volkoff, Melatonin is a key hormone involved in the photoperiodic signaling pathway. In both Memorial University of Newfoundland, teleosts and mammals, melatonin produced in the pineal gland at night is released into the Canada fl Hana Zemkova, blood and cerebrospinal uid, providing rhythmic information to the whole organism. Academy of Sciences of the Czech Melatonin acts via specific receptors, allowing the synchronization of daily and annual Republic (ASCR), Czechia physiological rhythms to environmental conditions. The pituitary gland, which produces *Correspondence: several hormones involved in a variety of physiological processes such as growth, Romain Fontaine [email protected] metabolism, stress and reproduction, is an important target of melatonin. Melatonin modulates pituitary cellular activities, adjusting the synthesis and release of the different Specialty section: pituitary hormones to the functional demands, which changes during the day, seasons This article was submitted to Neuroendocrine Science, and life stages. -
Behavioral and Feeding Ecology of a Small-Bodied Folivorous Primate (Lepilemur Leucopus)
Behavioral and Feeding Ecology of a Small-bodied Folivorous Primate (Lepilemur leucopus) Dissertation for the award of the degree “Doctor rerum naturalium” (Dr. rer. nat.) of the Georg-August-Universität Göttingen within the doctoral program Biology of the Georg-August University School of Science (GAUSS) submitted by Iris Dröscher from Scheifling, Austria Göttingen 2014 Thesis Committee: Prof. Dr. Peter M. Kappeler, Department of Sociobiology and Anthropology, Georg- August-Universität Göttingen, Behavioral Ecology and Sociobiology Unit, German Primate Center GmbH Prof. Dr. Eckhard W. Heymann, Department of Sociobiology and Anthropology, Georg- August-Universität Göttingen, Behavioral Ecology and Sociobiology Unit, German Primate Center GmbH Members of the Examination Board: Reviewer: Prof. Dr. Peter M. Kappeler Second Reviewer: Prof. Dr. Eckhard W. Heymann Further members of the Examination Board: Dr. Claudia Fichtel, Behavioral Ecology and Sociobiology Unit, German Primate Center GmbH Prof. Dr. Julia Ostner, Primate Social Evolution Group, Courant Research Centre Evolution of Social Behavior, Georg-August-Universität Göttingen Dr. Oliver Schülke, Primate Social Evolution Group, Courant Research Centre Evolution of Social Behavior, Georg-August-Universität Göttingen Dr. Dietmar Zinner, Cognitive Ethology Laboratory, German Primate Center GmbH Date of the oral examination: 12 December 2014 CONTENTS SUMMARY ………………………………………………………………………………1 ZUSAMMENFASSUNG …………………………………………………………………3 GENERAL INTRODUCTION …………………………………………………………...5 CHAPTER -
Primate Report 70 (2004).Pdf
C.C. Grüter and D. Zinner: Nested Social Systems in Primates NESTED SOCIETIES. CONVERGENT ADAPTATIONS OF BABOONS AND SNUB-NOSED MONKEYS? GRÜTER, C.C. AND ZINNER, D. Key Words: Rhinopithecus, Theropithecus, Papio, hamadryas baboon, gelada, snub- nosed monkeys, ecology, social system, evolution of multi-level society Abstract The social systems of geladas (Theropithecus gelada), hamadryas baboons (Papio hamadryas), black-and-white snub-nosed monkeys (Rhinopithecus bieti) and golden monkeys (Rhinopithecus roxellana) show some superficial similarities, mainly in their social organization. All four species live in a nested or multi-level society with one-male units (OMUs) as basal social entities (modules). Several OMUs group to- gether and constitute a second level of the social organization, the band. Aggrega- tions of OMUs and bands can comprise several hundred individuals and are among the largest primate groupings. However, despite those similarities, one can also ob- serve differences in the social organization, e.g. the existence of all-male units (AMUs) in geladas and snub-nosed monkeys, but not in hamadryas baboons, in the social structure, e.g. male-female relationships and the ontogeny of OMUs, and most likely also in the mating system. Furthermore, although the pattern of the social or- ganization is superficially similar, the phylogenetic processes that led to a nested so- cial system seem to be strikingly different. For geladas and hamadryas baboons, the origin of their system was most likely a multimale-multifemale group that split into OMUs, whereas in snub-nosed monkeys it seems more likely that the ancestral state were independent OMUs that grouped together. According to socio-ecological models of the evolution of primate social systems, ecological factors such as food-distribution and predation risk have major impacts on the spatio-temporal organization of primate females (and secondarily also of males) and their social relationships, and hence on the social system of a particular taxon. -
Melatonin Synthesis and Signaling for Seasonal Reproductive Timing By
Melatonin Synthesis and Signaling for Seasonal Reproductive Timing By Dax viviD A dissertation submitted in partial satisfaction of the requirements for the degree of Doctor of Philosophy in Integrative Biology in the Graduate Division of the University of California, Berkeley Committee in charge: Professor George E. Bentley, Chair Professor tyrone B hayes Professor Lance Kriegsfeld Professor Thomas Carlson Spring 2017 1 Abstract Melatonin Synthesis and Signaling for Seasonal Reproductive Timing by Dax viviD Doctor of Philosophy in Integrative Biology University of California, Berkeley Professor George Bentley, Chair This dissertation critically investigates research in melatonin across species and disciplines. It offers a broader perspective within the field of reproductive neuroendocrinology by integrating research in pharmacology, genetics, and evolution. The first chapter of this dissertation reviews melatonin administration techniques across fields. The timing, dose, and mode of administration is compared across species. This juxtaposition of previous protocols using melatonin administration illustrates the importance of context to help inform future experimental designs. Furthermore, details of the experiment that affect the findings are emphasized to improve replicability of melatonin administration studies. The second chapter focuses on melatonin synthesizing enzymes in two different songbirds. The zebra finch (Taeniopygia guttata) is an opportunistic breeder in the wild and uses primarily food and water availability to time reproduction (Perfito et al., 2007). The European starling (Sturnus vulgaris) is a photoperiodic breeder whose reproductive state relies on changes in day length (Gwinner, 1977). Melatonin- synthesizing enzymes were expressed in photoreceptive sites of the brain. Key melatonin- synthesizing enzymes were differentially expressed in the hypothalamus of the photoperiodic breeder at certain times of the year, and this expression was not analogously observed in the opportunistic breeder. -
Immunology of the Gray Short-Tailed Opossum During Pregnancy and Prenatal Development Victoria L
University of New Mexico UNM Digital Repository Biology ETDs Electronic Theses and Dissertations Spring 5-13-2017 Immunology of the gray short-tailed opossum during pregnancy and prenatal development Victoria L. Hansen University of New Mexico Follow this and additional works at: https://digitalrepository.unm.edu/biol_etds Part of the Bioinformatics Commons, Biology Commons, Evolution Commons, Immunology and Infectious Disease Commons, and the Laboratory and Basic Science Research Commons Recommended Citation Hansen, Victoria L.. "Immunology of the gray short-tailed opossum during pregnancy and prenatal development." (2017). https://digitalrepository.unm.edu/biol_etds/203 This Dissertation is brought to you for free and open access by the Electronic Theses and Dissertations at UNM Digital Repository. It has been accepted for inclusion in Biology ETDs by an authorized administrator of UNM Digital Repository. For more information, please contact [email protected]. Victoria L. Hansen Candidate Biology Department This dissertation is approved, and it is acceptable in quality and form for publication: Approved by the Dissertation Committee: Robert Miller , Chairperson Paul Samollow Stephen Stricker Christina Vesbach i IMMUNOLOGY OF THE GRAY SHORT-TAILED OPOSSUM DURING PREGNANCY AND PRENATAL DEVELOPMENT BY VICTORIA L. HANSEN B.A., Biology, University of Puget Sound, 2007 DISSERTATION Submitted in Partial Fulfillment of the Requirements for the Degree of Doctor of Philosophy Biology The University of New Mexico Albuquerque, New Mexico May, 2017 ii DEDICATION This dissertation is dedicated to my parents, Jeanne and Walt, who are unconditionally supportive and loving. Thank you, Mommy and Daddy. iii ACKNOWLEDGEMENTS Thank you to my mentor and PhD advisor, Dr. Rob Miller, and to my committee members Drs Paul Samollow, Stephen Stricker, and Christina Vesbach.