ME5312 Lifeform Final Report
Total Page:16
File Type:pdf, Size:1020Kb
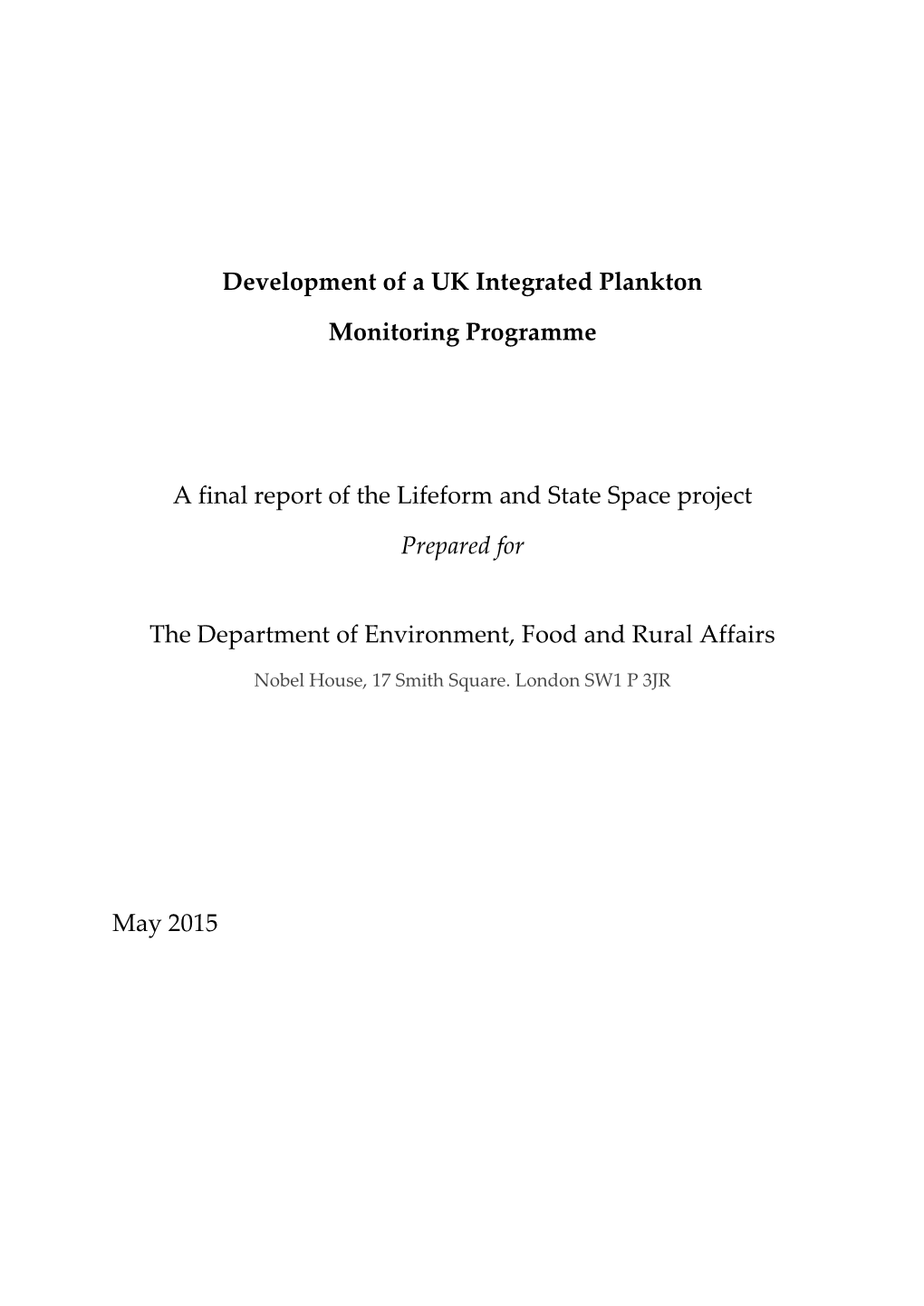
Load more
Recommended publications
-
Marine Plankton Diatoms of the West Coast of North America
MARINE PLANKTON DIATOMS OF THE WEST COAST OF NORTH AMERICA BY EASTER E. CUPP UNIVERSITY OF CALIFORNIA PRESS BERKELEY AND LOS ANGELES 1943 BULLETIN OF THE SCRIPPS INSTITUTION OF OCEANOGRAPHY OF THE UNIVERSITY OF CALIFORNIA LA JOLLA, CALIFORNIA EDITORS: H. U. SVERDRUP, R. H. FLEMING, L. H. MILLER, C. E. ZoBELL Volume 5, No.1, pp. 1-238, plates 1-5, 168 text figures Submitted by editors December 26,1940 Issued March 13, 1943 Price, $2.50 UNIVERSITY OF CALIFORNIA PRESS BERKELEY, CALIFORNIA _____________ CAMBRIDGE UNIVERSITY PRESS LONDON, ENGLAND [CONTRIBUTION FROM THE SCRIPPS INSTITUTION OF OCEANOGRAPHY, NEW SERIES, No. 190] PRINTED IN THE UNITED STATES OF AMERICA Taxonomy and taxonomic names change over time. The names and taxonomic scheme used in this work have not been updated from the original date of publication. The published literature on marine diatoms should be consulted to ensure the use of current and correct taxonomic names of diatoms. CONTENTS PAGE Introduction 1 General Discussion 2 Characteristics of Diatoms and Their Relationship to Other Classes of Algae 2 Structure of Diatoms 3 Frustule 3 Protoplast 13 Biology of Diatoms 16 Reproduction 16 Colony Formation and the Secretion of Mucus 20 Movement of Diatoms 20 Adaptations for Flotation 22 Occurrence and Distribution of Diatoms in the Ocean 22 Associations of Diatoms with Other Organisms 24 Physiology of Diatoms 26 Nutrition 26 Environmental Factors Limiting Phytoplankton Production and Populations 27 Importance of Diatoms as a Source of food in the Sea 29 Collection and Preparation of Diatoms for Examination 29 Preparation for Examination 30 Methods of Illustration 33 Classification 33 Key 34 Centricae 39 Pennatae 172 Literature Cited 209 Plates 223 Index to Genera and Species 235 MARINE PLANKTON DIATOMS OF THE WEST COAST OF NORTH AMERICA BY EASTER E. -
Article Is financed by CNRS-INSU
Clim. Past, 8, 415–431, 2012 www.clim-past.net/8/415/2012/ Climate doi:10.5194/cp-8-415-2012 of the Past © Author(s) 2012. CC Attribution 3.0 License. Climatically-controlled siliceous productivity in the eastern Gulf of Guinea during the last 40 000 yr X. Crosta1, O. E. Romero2, O. Ther1, and R. R. Schneider3 1CNRS/INSU, UMR5805, EPOC, Universite´ Bordeaux 1, Talence Cedex, France 2Instituto Andaluz de Ciencias de la Tierra (CSIC-UGR), 18100 Armilla-Granada, Spain 3Marine Klimaforschung, Institut fuer Geowissenschaften, CAU Kiel, Germany Correspondence to: X. Crosta ([email protected]) Received: 30 June 2011 – Published in Clim. Past Discuss.: 25 July 2011 Revised: 27 January 2012 – Accepted: 30 January 2012 – Published: 7 March 2012 Abstract. Opal content and diatom assemblages were anal- 1 Introduction ysed in core GeoB4905-4 to reconstruct siliceous produc- tivity changes in the eastern Gulf of Guinea during the last A substantial part of the ocean’s primary productivity is sup- 40 000 yr. Opal and total diatom accumulation rates pre- ported by diatoms (Treguer´ et al., 1995). At the global ocean sented low values over the considered period, except dur- scale, diatom productivity primarily depends on the avail- ing the Last Glacial Maximum and between 15 000 calendar ability of dissolved silica (DSi) as diatoms need DSi to build years Before Present (15 cal. ka BP) and 5.5 cal. ka BP, the their frustule. This explains why diatoms are dominant in the so-called African Humid Period, when accumulation rates of Southern Ocean, the North Pacific, coastal zones, and low- brackish and freshwater diatoms at the core site were high- latitude upwelling systems where high stocks of DSi prevail. -
Guinevere Installation Decommissioning Project Environmental Impact Assessment
GUINEVERE INSTALLATION DECOMMISSIONING PROJECT ENVIRONMENTAL IMPACT ASSESSMENT 2018 Guinevere Installation Decommissioning Project Environmental Impact Assessment PERENCO 3 Central Avenue | St Andrews Business Park Norwich | Norfolk | NR7 0HR PERENCO GUINEVERE INSTALLATION DECOMMISSIONING PROJECT ENVIRONMENTAL IMPACT ASSESSMENT Document Control Page Document Reference SN-PG-BX-AT-FD-000002 Number: Revision Record DATE REV NO. DESCRIPTION PREPARED CHECKED APPROVED 08/05/18 01 Draft for PUK review D Morgan G Jones (BMT G Nxumalo (BMT Cordah) Cordah) (Perenco) 04/06/18 02 Installation only D Morgan G Jones (BMT G Nxumalo (BMT Cordah) Cordah) (Perenco) 09/07/2018 03 BEIS comments G MacGlennon G Nxumalo G Nxumalo addressed (Perenco) (Perenco) (Perenco) PERENCO II GUINEVERE INSTALLATION DECOMMISSIONING PROJECT ENVIRONMENTAL IMPACT ASSESSMENT Standard Information Sheet Information Project Name Guinevere Installation Decommissioning Project Environmental Impact Assessment BEIS Reference No. n/a Type of Project Decommissioning Undertaker Name Perenco UK Limited Undertaker Address St Andrews Business Park, 3 Central Ave, Norwich, Norfolk, NR7 0HR Licensees/Owners Perenco Gas (UK) Limited (75%) Hansa Hydrocarbons (LAPS) Limited (25%) Short Description Perenco UK Limited propose to decommission the Guinevere Installation which is located within the UKCS Block 48/17b in the southern North Sea. Cessation of Production was approved by the Oil and Gas Authority in December 2016. This Environmental Impact Assessment has been prepared to support the Guinevere Installation Decommissioning Programme. The infrastructure which is included within the scope of the Guinevere Decommissioning Programme is summarised below: One (1) platform topside; One (1) four-legged jacket and four (4) piles; and Two (2) platform wells (48/17b-G1, 44/17b-G2). -
Strategic Environmental Assessment of the Mature Areas of the Offshore North Sea SEA 2
Report to the Department of Trade and Industry Strategic Environmental Assessment of the Mature Areas of the Offshore North Sea SEA 2 Consultation Document September 2001 2nd Strategic Environmental Assessment - Offshore North Sea CONTENTS Non-Technical Summary i 1 Introduction and Background 1 1.1 Introduction 1 1.2 Licensing context and SEA sequence 1 1.3 Scope and purpose of the SEA 2 1.4 Organisation of the consultation document 4 1.5 Supporting studies and documents 5 2 Strategic Environmental Assessment Process 7 2.1 Introduction 7 2.2 Overview of the SEA process 7 2.3 Scoping the SEA 8 2.4 Stakeholder dialogue session 8 2.5 Studies and surveys 9 2.6 Further consultation process 11 3 Regulatory Context 13 3.1 SEA Directive 13 3.2 Licensing 15 3.3 Control of operations 15 4 Activities 21 4.1 Introduction 21 4.2 Alternatives 21 4.3 Scenarios 21 4.4 Stages of activity 25 5 Physical and Chemical Environment 27 5.1 North Sea overview 27 5.2 Geology and substrates 27 5.3 Climate and meteorology 39 5.4 Oceanography and hydrography 40 5.5 Contamination of water and sediments 45 6 Ecology 51 6.1 North Sea overview 51 6.2 Plankton 52 6.3 Benthos 55 6.4 Cephalopods 66 6.5 Fish 68 6.6 Marine reptiles 78 6.7 Seabirds 79 6.8 Marine Mammals 90 7 Potential Offshore Conservation Sites 103 7.1 Overview 103 September 2001 i Contents 2nd Strategic Environmental Assessment - Offshore North Sea 7.2 Potential for sites within the SEA 2 areas 103 8 Existing Human Activity 109 8.1 Introduction 109 8.2 Oil and gas 109 8.3 Fisheries 110 8.4 Other uses of -
Phd Thesis the Taxa Are Listed Alphabetically Within the Bacteriastrum Genera and Each of the Chaetoceros Generic Subdivision (Subgenera)
FACULTY OF SCIENCE DEPARTMENT OF GEOLOGY INTERDISCIPLINARY DOCTORAL STUDY IN OCEANOLOGY Sunčica Bosak TAXONOMY AND ECOLOGY OF THE PLANKTONIC DIATOM FAMILY CHAETOCEROTACEAE (BACILLARIOPHYTA) FROM THE ADRIATIC SEA DOCTORAL THESIS Zagreb, 2013 PRIRODOSLOVNO-MATEMATIČKI FAKULTET GEOLOŠKI ODSJEK INTERDISCIPLINARNI DOKTORSKI STUDIJ IZ OCEANOLOGIJE Sunčica Bosak TAKSONOMIJA I EKOLOGIJA PLANKTONSKIH DIJATOMEJA IZ PORODICE CHAETOCEROTACEAE (BACILLARIOPHYTA) U JADRANSKOM MORU DOKTORSKI RAD Zagreb, 2013 FACULTY OF SCIENCE DEPARTMENT OF GEOLOGY INTERDISCIPLINARY DOCTORAL STUDY IN OCEANOLOGY Sunčica Bosak TAXONOMY AND ECOLOGY OF THE PLANKTONIC DIATOM FAMILY CHAETOCEROTACEAE (BACILLARIOPHYTA) FROM THE ADRIATIC SEA DOCTORAL THESIS Supervisors: Dr. Diana Sarno Prof. Damir Viličić Zagreb, 2013 PRIRODOSLOVNO-MATEMATIČKI FAKULTET GEOLOŠKI ODSJEK INTERDISCIPLINARNI DOKTORSKI STUDIJ IZ OCEANOLOGIJE Sunčica Bosak TAKSONOMIJA I EKOLOGIJA PLANKTONSKIH DIJATOMEJA IZ PORODICE CHAETOCEROTACEAE (BACILLARIOPHYTA) U JADRANSKOM MORU DOKTORSKI RAD Mentori: Dr. Diana Sarno Prof. dr. sc. Damir Viličić Zagreb, 2013 This doctoral thesis was made in the Division of Biology, Faculty of Science, University of Zagreb under the supervision of Prof. Damir Viličić and in one part in Stazione Zoologica Anton Dohrn in Naples, Italy under the supervision of Diana Sarno. The doctoral thesis was made within the University interdisciplinary doctoral study in Oceanology at the Department of Geology, Faculty of Science, University of Zagreb. The presented research was mainly funded by the Ministry of Science, Education and Sport of the Republic of Croatia Project No. 119-1191189-1228 and partially by the two transnational access projects (BIOMARDI and NOTCH) funded by the European Community – Research Infrastructure Action under the FP7 ‘‘Capacities’’ Specific Programme (Ref. ASSEMBLE grant agreement no. 227799). ACKNOWLEDGEMENTS ... to my Croatian supervisor and my boss, Prof. -
Using the PCI-LF - a Draft User Guide
Using the PCI-LF - a draft user guide Paul Tett Napier University, Edinburgh (v1.2) 2006 This document explains how to use the Matlab script PCI1ED2 and provides some explanatory material to help users of the PCI-LF tool. It is best read before using the script and tool, but you may go directly to the practical instructions (section 5) and run the script with the test data provided, reading the explanatory material afterwards. A licensed copy of Matlab version 7 or later is needed in order to run the script. Table of contents 1. Phytoplankton and sources of phytoplankton data.......................................................2 2. What is phytoplankton community structure and what is a PCI? .................................3 3. The PCI-LF explained.................................................................................................4 4. Lifeform theory ..........................................................................................................8 5. Using the Matlab script PCI1ED2 to calculate the PCI..............................................10 Overview..................................................................................................................10 Running the Matlab script.........................................................................................11 Data file preparation .................................................................................................12 Preparing the run control file ....................................................................................12 -
Index to Scientific Names
Index to Scientific Names References to main entries in bold-faced print, to illustrations in italics. Aaronsohnia 368 Adenoon 171 Alomia 556 Abrophyllaceae 57 Adenopappus 429 Alomiella 551 Abrophyllum 59 Adenophora 43 Alomiinae 552 A. ornans 59 Adenophyllum 424 Alseuosmia 10 Abrotanella 214 Adenostemma 518 A. banksii 9 A. linearis 215 A. viscosum 519 A. macrophylla 9 Acamptopappus 321 Adenostemmatinae 518 Alseuosmiaceae 3, 7 Acanthocephalus 184 Adenostyles 240 Alvordia 466 Acanthocladium 252 Adenothamnus 499 Amauria 509 Acanthodesmos 152 Aedesia 171 Amauriinae 509 Acantholepis 128 Aegialophila 145 Amauriopsis 435 Acanthospermum 488 Aegopordon 137 Ambassa 167 Acanthostyles 546 Aequatorium 223 Amberboa 142 Acanthotheca 245 A. jamesonii 223 Amblyocarpum 383 Acasma 132 A. subg. Praegynoxys 223 Amblyolepis 404 Achaetogeron 340 Aetheolaena 234 Amblyopappus 495 Achillea 364 Aetheopappus 140 Amblysperma 115 A. group 364 Aetheorhiza 190 Amboroa 571 Achnophora 295 Ageratella 555 Ambrosia 444 Achnopogon 98 Ageratina 167, 514 A. canescens 444 Achyrachaena 499 Ageratinae 520 A. polystachya 444 Achyrocline 252 Ageratinastrum 167 Ambrosiaceae 443 Achyrocome 263 Ageratum 522 Ambrosieae 443 Achyropappus 435 Agiabampoa 466 Ambrosiinae 441, 443 Achyroseris 198 Agnorhiza 464 Ameghinoa 106 Achyrothalamus 121 Agoseris 191 Amellus 291 Acicarpha 23 Agrianthus 542 A. asteroides 291 Acilepidopsis 166 A. group 542 Ammanthus 365 Acilepis 166 Ainsliaea 123 Ammobium 253 Acmella 471 Ajania 357 A. craspedioides 254 Acomis 252 A. group 357 Amolinia 572 Acosta 146 Ajaniopsis 357 Ampelaster 334 Acourtia 103 Akeassia 304 Ampherephis 160 Acrisione 223 Akylopsis 368 Amphiachyris 321 Acritopappus 522 Alatoseta 253 Amphidoxa 266 A. connatifolius 522 Albertinia 154 Amphiglossa 253 Acroclinium 279 Alcantara 163 Amphipappus 321 Acroptilon 143 Alciope 225 Amphoricarpos 132 Actinoseris 117 Aldama 465 Anacantha 137 Actionbole 252 Alepidocline 484 Anacyclus 364 A. -
Novosti Sist. Vyssh. Rast. 40
ÀËÔÀÂÈÒÍÛÉ ÓÊÀÇÀÒÅËÜ ÍÀÇÂÀÍÈÉ ÒÀÊÑÎÍΠ1 ALPHABEITICAL INDEX TO TAXA NAMES Æèðíûì øðèôòîì âûäåëåíû íàçâàíèÿ âïåðâûå îïèñûâàåìûõ òàêñîíîâ, íîâûå êîìáèíàöèè è íîâûå íàçâàíèÿ (nomina nova), à òàê- æå ñòðàíèöû, ãäå îáíàðîäîâàíû ýòè íàçâàíèÿ. Êðóæêîì (°) îòìå÷å- íû ñòðàíèöû, íà êîòîðûõ äàíû êàðòû àðåàëîâ, çâåçäî÷êîé (*) ñòðàíèöû, ãäå ïîìåùåíû èçîáðàæåíèÿ ñîîòâåòñòâóþùèõ òàêñîíîâ.  óêàçàòåëü íå âêëþ÷åíû íàçâàíèÿ, ñîäåðæàùèåñÿ â ñòàòüÿõ, ïîñâÿ- ùåííûõ òèïîâûì îáðàçöàì, êðîìå íàçâàíèé âïåðâûå îïèñûâàåìûõ òàêñîíîâ, íîâûõ êîìáèíàöèé è nomina nova. Acantholimon 224, 230 clavata 12, 13°, 32 alexandri 230 gigantea 11 × baubaschatense 224, 230, 231*, maritima 12 233 mertensii subsp. borealis 12 knorringianum 230, 232 stolonifera 12 ruprechtii 230, 232 subsp. straminea 12 Aegopordon 220223 straminea 12 berardioides 220223 tenuis 12 fontqueri 220, 221, 223 vulgaris 12 Agaloma purpurea 120 Alopecurus 25 Agropyron 7 sect. Alopecurium 25 cristatum 7 sect. Alopecurus 25 subsp. pectinatum 7 sect. Pseudophalaris 25 desertorum 7 aequalis 25 pectinatum 7 arundinaceus 25 pectiniforme 7 geniculatus 25 Agrostis 11 myosuroides 25 sect. Agraulus 12 pratensis 25 sect. Agrostis 11 ventricosus 25 sect. Trichodium 12 Anisantha 10 alba auct. 11 tectorum 10 borealis 12 Anthemis arvensis 241 canina 12 austriaca 241 capillaris 12, 31 Anthoxanthum 12 1 Óêàçàòåëü ñîñòàâëåí À. À. Ñâåòëîâîé. 345 alpinum 12 syzigachne 28, 32 subsp. borealis 15 Derderia 221 odoratum 12 Berardia 220 subsp. groenlandica 15 berardioides 222 subsp. alpinum 12 subacaulis 221 obtusata 15 Deschampsia 17 Apera 12 Blephariglottis 47, 48 purpurea 15, 31 cespitosa 17, 31 spica-venti 12 × channellii 48 subsp. langsdorffii 15 flexuosa 14 Apiaceae 184, 224 cristata × ciliaris 48 subsp. phragmitoides 15 Digraphis arundinacea 28 Arctopoa 236 × Blepharopsis 48 stricta 15 Dolomiaea 220 Arrhenatherum 14 vossii 48 Caprifoliaceae 197 elatius 14 Brachypodium 7 Carduus heterophyllus 241 Echinochloa 30 Artemisia 234, 240, 244 sect. -
Morphological Characters of Chaetoceros Lorenzianus (Bacillariophyceae) Isolated from North Arabian Sea After Tasman Spirit Oil Spill
ISSN (E): 2349 – 1183 ISSN (P): 2349 – 9265 3(3): 558–563, 2016 DOI: 10.22271/tpr.2016. v3.i3. 073 Research article Morphological characters of Chaetoceros lorenzianus (Bacillariophyceae) isolated from North Arabian Sea after Tasman Spirit oil spill Asma Tabassum1*, Hina Baig2 and Aliya Rehman1 1Department of Botany, University of Karachi, Karachi, Pakistan 2National Institute of Oceanography, Karachi, Pakistan *Corresponding Author: [email protected] [Accepted: 26 October 2016] Abstract: The present study investigated the morphology and taxonomy of marine centric diatom Chaetoceros lorenzianus from Karachi Harbor, Pakistan for the first time for the first time during the incident of Tasman Spirit Oil Spill (2003) in the area. Phytoplankton samples were collected from 5 different locations from the study area. Chaetoceros lorenzianus was found only at one station collected after Tasman Spirit Oil Spill. Moreover morphometric measurements of present record showed narrow range as compared to the records investigated by other workers. Keywords: Chaetoceros lorenzianus - Diatom - Phytoplankton - Tasman Spirit oil spill. [Cite as: Tabassum A, Baig H & Rehman A (2016) Morphological characters of Chaetoceros lorenzianus (Bacillariophyceae) isolated from North Arabian Sea after Tasman Spirit oil spill. Tropical Plant Research 3(3): 558–563] INTRODUCTION Phytoplankton are the major primary producers of marine and fresh water environment (Baliarsingh et al. 2012). Among phytoplankton, the Bacillariophyta (diatoms) contributes at least 40% of the global annual primary productivity (Field et al. 1998). These diatoms are ubiquitous occurrence in marine environment (Sunesen et al. 2008). Genus Chaetoceros Ehrenberg is considered as most diverse and wide spread centric diatom (Cupp 1943, Rines 1999, Hasle & Syvertsen 1997). -
Arctium-Cousinia
Evolució, filogènia i sistemàtica del complex Arctium-Cousinia TESI DOCTORAL Sara López Viñallonga Barcelona 2009 UNIVERSITAT DE BARCELONA FACULTAT DE BIOLOGIA Departament de Biologia Vegetal, Unitat de Botànica Programa de Doctorat: Biologia Vegetal Bienni: 2002-2004 INSTITUT BOTÀNIC DE BARCELONA (CSIC-ICUB) EVOLUCIÓ, FILOGÈNIA I SISTEMÀTICA DEL COMPLEX ARCTIUM-COUSINIA Memòria presentada per Sara López Viñallonga per a optar al títol de Doctor per la Universitat de Barcelona Amb el vist-i-plau dels directors de tesi: Dra. Núria Garcia Jacas Dr. Alfonso Susanna de la Serna i del tutor de tesi: Dr. Ramon M. Masalles i Saumell Sara López Viñallonga Barcelona, 2009 Penseu que el mirall de la veritat s’esmicolà a l’origen en fragments petitíssims, i cada un dels trossos recull tanmateix una engruna d’autèntica llum. Salvador Espriu Agraïments Primer de tot vull agrair als meus directors de tesi, la doctora Núria Garcia i el doctor Alfonso Susanna, que em fitxessin per aquest projecte que m’ha convertit en una friki de la sistemàtica. El vostre suport incondicional i la vostra ajuda han estat essencials per dur a terme aquest treball; fins i tot us he fet treballar els caps de setmana! Núria, gràcies per salvar-me del “fer per fer”. Alfonso, no te tendré en cuenta que me hayas metido en este berenjenal si me perdonas el pateo buscando la fedtschenkoana! La meva màxima gratitud també és per al Dr. Masalles que en tot moment ha estat disponible per ajudar-me amb la paperassa i m’ha animat sempre que l’he anat a veure. -
Inspection and Cover Spraying of Nursery Plants to WA
DEPARTMENT OF PRIMARY INDUSTRIES Inspection and Cover Spraying of Nursery Plants to WA ICA-52 – Treatment for Currant Lettuce Aphid Version 1.0 ICA-52 INSPECTION AND COVER SPRAYING PLANTS REVISION REGISTER Date of Issue Amendment Details 05/08/2005 First Issue © State Government of Victoria, Department of Primary Industries 2005. This publication is copyright. No part may be reproduced by any process except in accordance with the provisions of the Copyright Act 1968. ISBN 1 74146 438 2 For further clarification or advice on any area covered in this publication, please contact Plant Standards on (03) 9210 9390 or at www.dpi.vic.gov.au/psb Authorised by the Victorian Government Department of Primary Industries Plant Standards 621 Burwood Highway, Knoxfield, Victoria 3156 Disclaimer This publication may be of assistance to you but the State of Victoria and its employees do not guarantee that the publication is without flaw of any kind or is wholly appropriate for your particular purposes and therefore disclaims all liability for any error, loss or other consequence which may arise from you relying on any information in this publication. Version 1.0 (Aug 2005) Page 2 of 24 ICA-52 INSPECTION AND COVER SPRAYING PLANTS Contents 1. Purpose .............................................................................. 5 2. Scope.................................................................................. 5 3. References .......................................................................... 5 4. Definitions ......................................................................... -
Îáçîð Ðîäà Aegopordon Boiss. (Asteraceae)
Î. Â. ×åðíåâà O. Tscherneva (Äæåôôðè, 2002). Åäèíñòâåííûé âèä ýòîãî ðîäà B. subacaulis Vill. èìååò ëèøü ãàáèòóàëüíîå ñõîäñòâî ñ Aegopordon berardioides. ÎÁÇÎÐ ÐÎÄÀ AEGOPORDON BOISS. Ïî ìîðôîëîãè÷åñêèì ïðèçíàêàì ðîä Aegopordon íàèáîëåå áëèçîê (ASTERACEAE) ê ðîäó Jurinea. Ðîäîâàÿ ñàìîñòîÿòåëüíîñòü Aegopordon äîëãîå âðåìÿ SYNOPSIS OF THE GENUS AEGOPORDON BOISS. íå áûëà ïðèíÿòà, è åãî âêëþ÷àëè â ðîäû Jurinea, Derderia è Ono- (ASTERACEAE) pordon.  ïîñëåäíèå ãîäû ñàìîñòîÿòåëüíîñòü ýòîãî ðîäà ïðèçíàíà ìíîãèìè àâòîðàìè (Rechinger, 1979). Áîòàíè÷åñêèé èíñòèòóò èì. Â. Ë. Êîìàðîâà ÐÀÍ, Ãåðáàðèé âûñøèõ ðàñòåíèé Îòëè÷èòåëüíûå ïðèçíàêè ðîäà Aegopordon îò áëèçêîðîäñòâåííî- 197376, Ñàíêò-Ïåòåðáóðã, óë. Ïðîôåññîðà Ïîïîâà, 2 ãî ðîäà Jurinea ïðèâåäåíû â òàáëèöå (ñì.). [email protected] Ïðè êðèòè÷åñêîì èçó÷åíèè âèäîâ ðîäà Jurinea ñ Ïèðåíåéñêîãî Ïðèâîäèòñÿ òàêñîíîìè÷åñêèé îáçîð ðîäà Aegopordon Boiss., âêëþ÷àþùå- ïîëóîñòðîâà, ÷òî ÿâëÿåòñÿ âåñüìà àêòóàëüíîé çàäà÷åé ïðè ñîçäàíèè ãî 2 âèäà: A. berardioides Boiss. è A. fontqueri (Cuatrec.) Tschern. comb. nova. ñîâðåìåííîé ìîíîãðàôèè ýòîãî òàêñîíà, ìû îáíàðóæèëè î÷åíü îðè- Ïîêàçàíû îòëè÷èòåëüíûå ïðèçíàêè ðîäà Aegopordon îò áëèçêîðîäñòâåííîãî ãèíàëüíûé âèä J. fontqueri Cuatrec., äåòàëüíî îïèñàííûé ñ þãà ðîäà Jurinea Cass. Ïèðåíåéñêîãî ïîëóîñòðîâà (ïðîâèíöèÿ Jaén) (Cuatrecasas, 1927). Àâ- Êëþ÷åâûå ñëîâà: Aegopordon, Jurinea, Asteraceae. òîð äàííîãî âèäà ñ íåêîòîðûì ñîìíåíèåì îòíåñ åãî ê ñåêöèè  ñòàòüå ïðåäñòàâëåí äåòàëüíûé òàêñîíîìè÷åñêèé àíàëèç ðîäà Aegopordon ðîäà Jurinea. Ïðè îïèñàíèè J. fontqueri Êóàòðåêàñàñ íåî- Aegopordon Boiss., ïðåäñòàâèòåëÿ ïîäñåìåéñòâà Carduoideae òðèáû äíîêðàòíî óêàçûâàåò íà òî, ÷òî ýòîò âèä ãàáèòóñîì î÷åíü íàïîìèíàåò Cynareae ïîäòðèáû Carduinae. Ýòîò ðîä áëèçîê ê ðîäàì Saussurea DC. Aegopordon berardioides, èñïîëüçóÿ äëÿ ïîñëåäíåãî ñîîòâåòñòâóþùåå è Jurinea Cass. è îáðàçóåò ñ íèìè îñîáóþ êëàäó «ðîäñòâà Jurinea Cass., ðîäîâîå íàçâàíèå, êîòîðîå, îäíàêî, îí òàê è íå ïðèíÿë.