A Total-Evidence Dated Phylogeny of Echinoids and the Evolution of Body
Total Page:16
File Type:pdf, Size:1020Kb
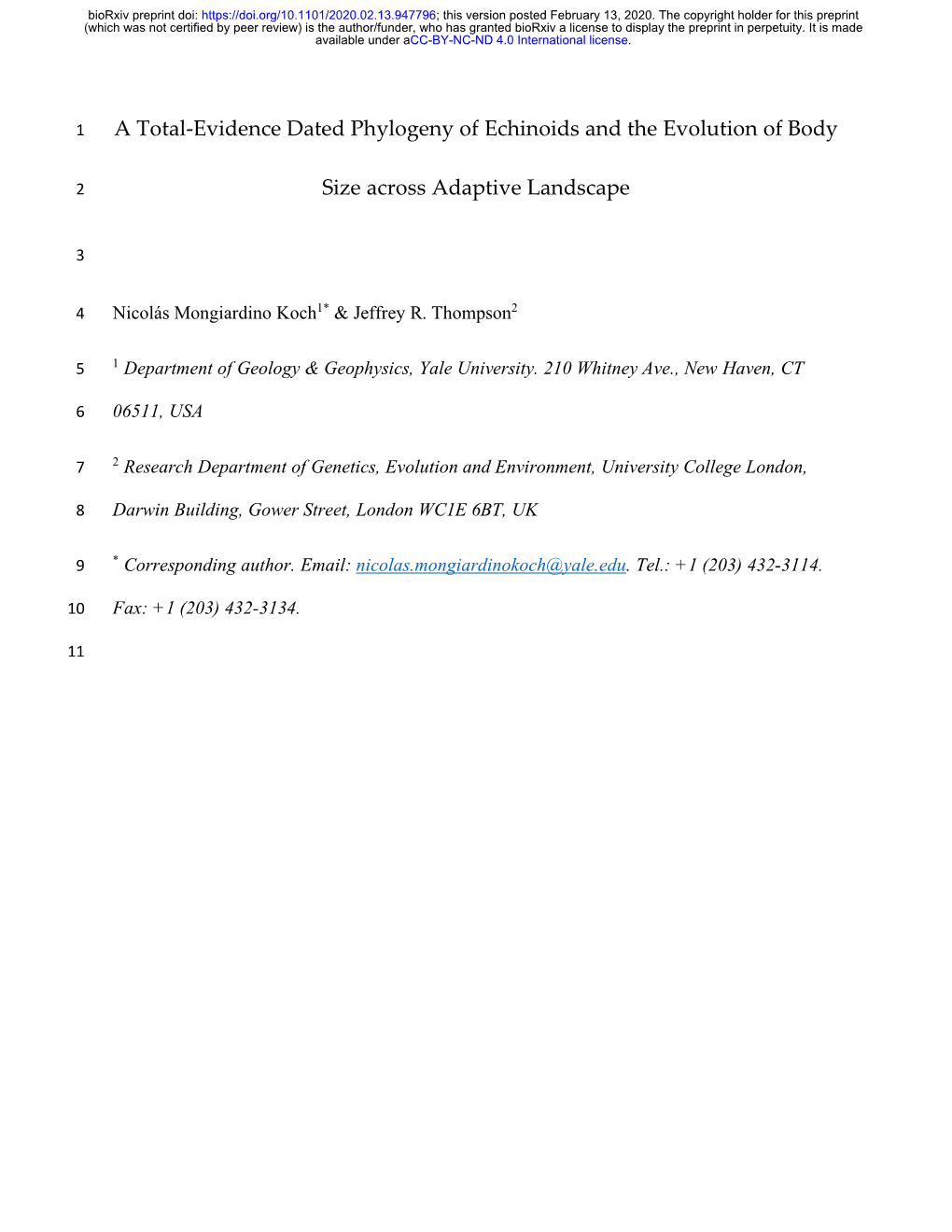
Load more
Recommended publications
-
Grazing by the Sea Urchins Arbacia Lixula L. and Paracentrotus Lividus Lam. in the Northwest Mediterranean
Journal of Experimental Marine Biology and Ecology, L 241 (1999) 81±95 Grazing by the sea urchins Arbacia lixula L. and Paracentrotus lividus Lam. in the Northwest Mediterranean Fabio Bulleri, Lisandro Benedetti-Cecchi* , Francesco Cinelli Dipartimento di Scienze dell'Uomo e dell'Ambiente via A. Volta 6, 56126 Pisa, Italy Received 2 October 1998; received in revised form 12 April 1999; accepted 5 May 1999 Abstract The sea urchins Arbacia lixula and Paracentrotus lividus are common on shallow subtidal reefs in the Mediterranean. Previous studies on the ecology of these species reported that P. lividus is generally more abundant on horizontal or gently sloping substrata, where it forages mainly on erect algae. In contrast, A. lixula is more common on vertical substrata and it is considered a main grazer of encrusting coralline algae. Observations on some rocky shores in the Ligurian sea indicated that P. lividus occurs mainly in crevices at the bottom of the vertical walls, and that neither species is present on horizontal or sub-horizontal substrata. In this study we investigated the distribution and abundance of the two species of sea urchins on vertical substrata at different spatial scales and through time. A ®eld experiment was used to test whether A. lixula constrained the distribution of P. lividus on vertical substrata, and to test for the predicted differences in the effects of the 2 species on assemblages of algae and invertebrates. Four treatments were used: (1) control (sea urchins left untouched); (2) A. lixula removed, P. lividus present; (3) A. lixula present, P. lividus removed, and (4) both species removed. -
Males and Females Gonad Fatty Acids of the Sea Urchins and (Echinodermata) Inés Martínez-Pita, Francisco J
Males and females gonad fatty acids of the sea urchins and (Echinodermata) Inés Martínez-Pita, Francisco J. García, María-Luisa Pita To cite this version: Inés Martínez-Pita, Francisco J. García, María-Luisa Pita. Males and females gonad fatty acids of the sea urchins and (Echinodermata). Helgoland Marine Research, Springer Verlag, 2009, 64 (2), pp.135-142. 10.1007/s10152-009-0174-7. hal-00535207 HAL Id: hal-00535207 https://hal.archives-ouvertes.fr/hal-00535207 Submitted on 11 Nov 2010 HAL is a multi-disciplinary open access L’archive ouverte pluridisciplinaire HAL, est archive for the deposit and dissemination of sci- destinée au dépôt et à la diffusion de documents entific research documents, whether they are pub- scientifiques de niveau recherche, publiés ou non, lished or not. The documents may come from émanant des établissements d’enseignement et de teaching and research institutions in France or recherche français ou étrangers, des laboratoires abroad, or from public or private research centers. publics ou privés. Helgol Mar Res (2010) 64:135–142 DOI 10.1007/s10152-009-0174-7 ORIGINAL ARTICLE Males and females gonad fatty acids of the sea urchins Paracentrotus lividus and Arbacia lixula (Echinodermata) Inés Martínez-Pita · Francisco J. García · María-Luisa Pita Received: 25 May 2009 / Revised: 18 September 2009 / Accepted: 24 September 2009 / Published online: 15 October 2009 © Springer-Verlag and AWI 2009 Abstract The aim of this study was to analyze male and 20:5n-3 and 22:6n-3 found in males and females of the female gonad fatty acids of two sea urchin species, Para- Mediterranean specimens compared to those of the Atlantic centrotus lividus and Arbacia lixula, from the south coast coast. -
SI Appendix for Hopkins, Melanie J, and Smith, Andrew B
Hopkins and Smith, SI Appendix SI Appendix for Hopkins, Melanie J, and Smith, Andrew B. Dynamic evolutionary change in post-Paleozoic echinoids and the importance of scale when interpreting changes in rates of evolution. Corrections to character matrix Before running any analyses, we corrected a few errors in the published character matrix of Kroh and Smith (1). Specifically, we removed the three duplicate records of Oligopygus, Haimea, and Conoclypus, and removed characters C51 and C59, which had been excluded from the phylogenetic analysis but mistakenly remain in the matrix that was published in Appendix 2 of (1). We also excluded Anisocidaris, Paurocidaris, Pseudocidaris, Glyphopneustes, Enichaster, and Tiarechinus from the character matrix because these taxa were excluded from the strict consensus tree (1). This left 164 taxa and 303 characters for calculations of rates of evolution and for the principal coordinates analysis. Other tree scaling methods The most basic method for scaling a tree using first appearances of taxa is to make each internal node the age of its oldest descendent ("stand") (2), but this often results in many zero-length branches which are both theoretically questionable and in some cases methodologically problematic (3). Several methods exist for modifying zero-length branches. In the case of the results shown in Figure 1, we assigned a positive length to each zero-length branch by having it share time equally with a preceding, non-zero-length branch (“equal”) (4). However, we compared the results from this method of scaling to several other methods. First, we compared this with rates estimated from trees scaled such that zero-length branches share time proportionally to the amount of character change along the branches (“prop”) (5), a variation which gave almost identical results as the method used for the “equal” method (Fig. -
Fossil Spatangoid Echinoids of Cuba
SMITHSONIAN CONTRIBUTIONS TO PALEOBIOLOGY • NUMBER 55 Fossil Spatangoid Echinoids of Cuba Porter M. Kier SMITHSONIAN INSTITUTION PRESS City of Washington 1984 ABSTRACT Kier, Porter M. Fossil Spatangoid Echinoids of Cuba. Smithsonian Contributions to Paleobiology, number 55, 336 pages, frontispiece, 45 figures, 90 plates, 6 tables, 1984.—The fossil spatangoid echinoids of Cuba are described based for the most part on specimens in the Sanchez Roig Collection. Seventy-nine species are recognized including 10 from the Late Cretaceous, 36 from the Eocene, 20 from the Oligocene-Miocene, 11 from the Miocene, and 2 of uncertain age. Three of the Eocene species are new: Schizas ter forme III, Linthia monteroae, and Antillaster albeari. A new genus of schizasterid is described, Caribbaster, with the Eocene Prenaster loveni Cotteau as the type-species. A new Asterostoma, A. pawsoni, is described from the Eocene of Jamaica. The Eocene age of the Cuban echinoid-bearing localities is confirmed by the presence outside Cuba of many ofthe same species in beds dated on other fossils. Some evidence supports the Miocene determinations, but the echinoids are of little assistance in resolving the question whether the Cuban beds attributed to the Oligocene are Oligocene or Miocene. Cuban, and in general, the Caribbean Tertiary echinoid faunas are distinct from those in Europe and the Mediterranean. Many genera are confined to the Caribbean. The Cuban fauna is also different from that found nearby in Florida. This difference may be due to a suggested greater depth of water in Cuba. Se describen los equinoideos espatangoideos de Cuba, incluyendo los es- pecimenes de la Coleccion Sanchez Roig. -
Echinodermata: Echinoidea) and Other New Records from the Early Paleocene Bruderndorf Formation in Austria 309-325
ZOBODAT - www.zobodat.at Zoologisch-Botanische Datenbank/Zoological-Botanical Database Digitale Literatur/Digital Literature Zeitschrift/Journal: Annalen des Naturhistorischen Museums in Wien Jahr/Year: 2004 Band/Volume: 106A Autor(en)/Author(s): Kroh Andreas Artikel/Article: Cottreaucorys kollmanni n. sp. (Echinodermata: Echinoidea) and other new records from the Early Paleocene Bruderndorf Formation in Austria 309-325 ©Naturhistorisches Museum Wien, download unter www.biologiezentrum.at Ann. Naturhist. Mus. Wien 106 A 309–325 Wien, November 2004 Cottreaucorys kollmanni n. sp. (Echinodermata: Echinoidea) and other new records from the Early Paleocene Bruderndorf Formation in Austria By Andreas KROH1 (With 3 textfigures and 2 plates) Manuscript submitted on 8 March 2004, the revised manuscript on 6 May 2004 Zusammenfassung Diese Arbeit stellt eine Ergänzung zur Revision der Echinidenfauna des Oberen Danium der Bruderndorf- Formation (Waschberg Zone, nordöstliches Österreich; siehe KROH 2001) dar. Cottreaucorys kollmanni n. sp., eine neuer Vertreter der Aeropsidae, einer Gruppe von seltenen Tiefwasser-Spatangiden, wird be- schrieben. Weiters konnten die Arten Procassidulus cf. elongatus und Galeaster bertrandi erstmals aus Österreich nachgewiesen werden. Zusätzlich wird neues, besser erhaltenes Material von Adelopneustes montainvillensis und Linthia houzeaui abgebildet, Arten die ursprünglich anhand von relativ schlecht er- haltenem Material nachgewiesen wurden. Schlüsselwörter: Echinoidea, Echinodermata, Danium, Paläogen, Österreich, Cottreaucorys kollmanni n. sp. Abstract This paper concerns additional new records of echinoids from the Late Danian Bruderndorf Formation out- cropping in the Waschberg Zone in North-eastern Austria. Cottreaucorys kollmanni n.sp. is established for a new small aeropsid, a group of rare deep-water spatangoids. Furthermore, the species Procassidulus cf. elongatus and Galeaster bertrandi are recorded for the first time from Austria. -
Phylogenomic Analyses of Echinoid Diversification Prompt a Re
bioRxiv preprint doi: https://doi.org/10.1101/2021.07.19.453013; this version posted August 4, 2021. The copyright holder for this preprint (which was not certified by peer review) is the author/funder, who has granted bioRxiv a license to display the preprint in perpetuity. It is made available under aCC-BY-NC-ND 4.0 International license. 1 Phylogenomic analyses of echinoid diversification prompt a re- 2 evaluation of their fossil record 3 Short title: Phylogeny and diversification of sea urchins 4 5 Nicolás Mongiardino Koch1,2*, Jeffrey R Thompson3,4, Avery S Hatch2, Marina F McCowin2, A 6 Frances Armstrong5, Simon E Coppard6, Felipe Aguilera7, Omri Bronstein8,9, Andreas Kroh10, Rich 7 Mooi5, Greg W Rouse2 8 9 1 Department of Earth & Planetary Sciences, Yale University, New Haven CT, USA. 2 Scripps Institution of 10 Oceanography, University of California San Diego, La Jolla CA, USA. 3 Department of Earth Sciences, 11 Natural History Museum, Cromwell Road, SW7 5BD London, UK. 4 University College London Center for 12 Life’s Origins and Evolution, London, UK. 5 Department of Invertebrate Zoology and Geology, California 13 Academy of Sciences, San Francisco CA, USA. 6 Bader International Study Centre, Queen's University, 14 Herstmonceux Castle, East Sussex, UK. 7 Departamento de Bioquímica y Biología Molecular, Facultad de 15 Ciencias Biológicas, Universidad de Concepción, Concepción, Chile. 8 School of Zoology, Faculty of Life 16 Sciences, Tel Aviv University, Tel Aviv, Israel. 9 Steinhardt Museum of Natural History, Tel-Aviv, Israel. 10 17 Department of Geology and Palaeontology, Natural History Museum Vienna, Vienna, Austria 18 * Corresponding author. -
For Peer Review
Page 1 of 40 Geological Journal Page 1 of 32 1 2 3 Neogene echinoids from the Cayman Islands, West Indies: regional 4 5 6 implications 7 8 9 10 1 2 3 11 STEPHEN K. DONOVAN *, BRIAN JONES and DAVID A. T. HARPER 12 13 14 15 1Department of Geology, Naturalis Biodiversity Center, Leiden, the Netherlands 16 17 2Department of Earth and Atmospheric Sciences, University of Alberta, Edmonton, Canada, T6G 2E3 18 For Peer Review 19 3 20 Department of Earth Sciences, Durham University, Durham, UK 21 22 23 24 25 26 27 28 29 30 31 32 33 34 35 36 37 38 39 40 41 42 43 44 45 46 47 48 *Correspondence to: S. K. Donovan, Department of Geology, Naturalis Biodiversity Center, 49 50 Darwinweg 2, 2333 CR Leiden, the Netherlands. 51 52 E-mail: [email protected] 53 54 55 56 57 58 59 60 http://mc.manuscriptcentral.com/gj Geological Journal Page 2 of 40 Page 2 of 32 1 2 3 The first fossil echinoids are recorded from the Cayman Islands. A regular echinoid, Arbacia? sp., the 4 5 spatangoids Brissus sp. cf. B. oblongus Wright and Schizaster sp. cf. S. americanus (Clark), and the 6 7 clypeasteroid Clypeaster sp. are from the Middle Miocene Cayman Formation. Test fragments of the 8 9 mellitid clypeasteroid, Leodia sexiesperforata (Leske), are from the Late Pleistocene Ironshore 10 11 Formation. Miocene echinoids are preserved as (mainly internal) moulds; hence, all species are left 12 13 14 in open nomenclature because of uncertainties regarding test architecture. -
The Carboniferous Evolution of Nova Scotia
Downloaded from http://sp.lyellcollection.org/ by guest on September 27, 2021 The Carboniferous evolution of Nova Scotia J. H. CALDER Nova Scotia Department of Natural Resources, PO Box 698, Halifax, Nova Scotia, Canada B3J 2T9 Abstract: Nova Scotia during the Carboniferous lay at the heart of palaeoequatorial Euramerica in a broadly intermontane palaeoequatorial setting, the Maritimes-West-European province; to the west rose the orographic barrier imposed by the Appalachian Mountains, and to the south and east the Mauritanide-Hercynide belt. The geological affinity of Nova Scotia to Europe, reflected in elements of the Carboniferous flora and fauna, was mirrored in the evolution of geological thought even before the epochal visits of Sir Charles Lyell. The Maritimes Basin of eastern Canada, born of the Acadian-Caledonian orogeny that witnessed the suture of Iapetus in the Devonian, and shaped thereafter by the inexorable closing of Gondwana and Laurasia, comprises a near complete stratal sequence as great as 12 km thick which spans the Middle Devonian to the Lower Permian. Across the southern Maritimes Basin, in northern Nova Scotia, deep depocentres developed en echelon adjacent to a transform platelet boundary between terranes of Avalon and Gondwanan affinity. The subsequent history of the basins can be summarized as distension and rifting attended by bimodal volcanism waning through the Dinantian, with marked transpression in the Namurian and subsequent persistence of transcurrent movement linking Variscan deformation with Mauritainide-Appalachian convergence and Alleghenian thrusting. This Mid- Carboniferous event is pivotal in the Carboniferous evolution of Nova Scotia. Rapid subsidence adjacent to transcurrent faults in the early Westphalian was succeeded by thermal sag in the later Westphalian and ultimately by basin inversion and unroofing after the early Permian as equatorial Pangaea finally assembled and subsequently rifted again in the Triassic. -
Biodiversidad De Los Equinodermos (Echinodermata) Del Mar Profundo Mexicano
Biodiversidad de los equinodermos (Echinodermata) del mar profundo mexicano Francisco A. Solís-Marín,1 A. Laguarda-Figueras,1 A. Durán González,1 A.R. Vázquez-Bader,2 Adolfo Gracia2 Resumen Nuestro conocimiento de la diversidad del mar profundo en aguas mexicanas se limita a los escasos estudios existentes. El número de especies descritas es incipiente y los registros taxonómicos que existen provienen sobre todo de estudios realizados por ex- tranjeros y muy pocos por investigadores mexicanos, con los cuales es posible conjuntar algunas listas faunísticas. Es importante dar a conocer lo que se sabe hasta el momen- to sobre los equinodermos de las zonas profundas de México, información básica para diversos sectores en nuestro país, tales como los tomadores de decisiones y científicos interesados en el tema. México posee hasta el momento 643 especies de equinoder- mos reportadas en sus aguas territoriales, aproximadamente el 10% del total de las especies reportadas en todo el planeta (~7,000). Según los registros de la Colección Nacional de Equinodermos (ICML, UNAM), la Colección de Equinodermos del “Natural History Museum, Smithsonian Institution”, Washington, DC., EUA y la bibliografía revisa- 1 Colección Nacional de Equinodermos “Ma. E. Caso Muñoz”, Laboratorio de Sistemá- tica y Ecología de Equinodermos, Instituto de Ciencias del Mar y Limnología (ICML), Universidad Nacional Autónoma de México (UNAM). Apdo. Post. 70-305, México, D. F. 04510, México. 2 Laboratorio de Ecología Pesquera de Crustáceos, Instituto de Ciencias del Mar y Lim- nología (ICML), (UNAM), Apdo. Postal 70-305, México D. F., 04510, México. 215 da, existen 348 especies de equinodermos que habitan las aguas profundas mexicanas (≥ 200 m) lo que corresponde al 54.4% del total de las especies reportadas para el país. -
Geology and Biology of North Atlantic Deep-Sea Cores
If :you do not need this publication after it has served your purpose, please return it to the Geological Surve:y, using the official mailing label at the end II UNITED STATES DEPARTMENT OF THE INTERIOR GEOLOGY AND BIOLOGY OF NORTH ATLANTIC DEEP-SEA CORES Part 5. MOLLUSCA Part 6. ECHINODERMATA Part 7. MISCELLANEOUS FOSSILS AND SIGNIFICANCE OF FAUNAL DISTRIBUTION GEOLOGICAL SURVEY PROFESSIONAL PAPER 196-D UNITED STATES DEPARTMENT OF THE INTERIOR Harold L. Ickes, Secretary GEOLOGICAL SURVEY W. C. Mendenhall, Director Professional Paper 196-D GEOLOGY AND BIOLOGY OF NORTH ATLANTIC DEEP-SEA CORES BETWEEN NEWFOUNDLAND AND IRELAND PART 5. MOLLUSCA By HARALD A. REHDER PART 6. ECHINODERMATA By AusTIN H. CLARK PART 7. MISCELLANEOUS FOSSILS AND SIGNIFICANCE OF FAUNAL DISTRIBUTION By LLOYD G. HENBEST UNITED STATES GOVERNMENT PRINTING OFFICE WASHINGTON : 1942 For sale by the Supel"intendent of Documents, Washington, D. C. - ---- Price 30 cents CONTENTS Page .Page Outline of the complete report _______________________ _ v- Association of the species on the present ocean Summary of the complete ~eport _____________________ _ VI bottom---------------~---------------------- 116 Foreword, by C. S. Piggot_ _________________________ _ "1 Relation of species to distance below top of core ___ _ 117 General introduction, by W. H. Bradley ______________ _ XIII Part. 7. Miscellaneous fossils and significance of faunal Significance of the investigation _________________ _ XIII distribution, by Lloyd (}. HenbesL _________ _ 119 Location of the core stations ____________________ _ XIV Introduction __________________________________ _ 119 Personnel and composition of the report __________ _ XIV Methods of preparation and study _______________ _ 119' Methods of sampling and examination ____________ _ XIV Notes on the groups of fossils ___________________ . -
The Irregular Sea Urchins (Echinodermata: Echinoidea)
Zoological Studies 39(3): 250-265 (2000) The Irregular Sea Urchins (Echinodermata: Echinoidea) from Taiwan, with Descriptions of Six New Records Shyh-Min Chao Division of Zoology, National Museum of Natural Science, Taichung, Taiwan 404, R.O.C. Tel: 886-4-3226940 ext. 502. Fax: 886-4-3232146. (Accepted March 27, 2000) Shyh-Min Chao (2000) The irregular sea urchins (Echinodermata: Echinoidea) from Taiwan, with descriptions of six new records. Zoological Studies 39(3): 250-265. Taiwans irregular sea urchin fauna now comprises 19 valid species in 11 families. New records include Fibularia ovulum Lamarck (Fibulariidae), Astriclypeus manni Verrill (Astriclypeidae), Linopneustes sp. (Palaeopneustidae), Schizaster lacunosus (Linnaeus) (Schizasteridae), Brissus latecarinatus (Leske), and Rhynobrissus pyramidalis A. Agassiz (Brissidae). Species accounts and figures of 14 species collected by the author are presented. Key words: Sea urchins, Irregular urchins, Echinoderms, Taiwan, Taxonomy. Sea urchins may be either regular or irregular. Regular urchins have an almost spherical symmetry. Irregular urchins display varying degrees of bilateral symmetry. They are common macrobenthic organ- isms along the coasts of Taiwan. However, only a few papers dealing with them have been published (Tokunaga 1900, Ohshima 1927, Hayasaka 1948, Peng and Tiao 1971, Chen and Chang 1981, Shigei 1981, Wang 1984) on the ecology and systematics of these animals from the waters of Taiwan. The regu- lar sea urchins from Taiwan have been revised by Chen and Chang (1981). However, there has been no study of the irregular urchins from Taiwan since Hayasaka (1948) except for a new species, Tai- wanaster mai (now Sinaechinocyamus mai), de- scribed by Wang (1984). -
An Annotated Checklist of the Marine Macroinvertebrates of Alaska David T
NOAA Professional Paper NMFS 19 An annotated checklist of the marine macroinvertebrates of Alaska David T. Drumm • Katherine P. Maslenikov Robert Van Syoc • James W. Orr • Robert R. Lauth Duane E. Stevenson • Theodore W. Pietsch November 2016 U.S. Department of Commerce NOAA Professional Penny Pritzker Secretary of Commerce National Oceanic Papers NMFS and Atmospheric Administration Kathryn D. Sullivan Scientific Editor* Administrator Richard Langton National Marine National Marine Fisheries Service Fisheries Service Northeast Fisheries Science Center Maine Field Station Eileen Sobeck 17 Godfrey Drive, Suite 1 Assistant Administrator Orono, Maine 04473 for Fisheries Associate Editor Kathryn Dennis National Marine Fisheries Service Office of Science and Technology Economics and Social Analysis Division 1845 Wasp Blvd., Bldg. 178 Honolulu, Hawaii 96818 Managing Editor Shelley Arenas National Marine Fisheries Service Scientific Publications Office 7600 Sand Point Way NE Seattle, Washington 98115 Editorial Committee Ann C. Matarese National Marine Fisheries Service James W. Orr National Marine Fisheries Service The NOAA Professional Paper NMFS (ISSN 1931-4590) series is pub- lished by the Scientific Publications Of- *Bruce Mundy (PIFSC) was Scientific Editor during the fice, National Marine Fisheries Service, scientific editing and preparation of this report. NOAA, 7600 Sand Point Way NE, Seattle, WA 98115. The Secretary of Commerce has The NOAA Professional Paper NMFS series carries peer-reviewed, lengthy original determined that the publication of research reports, taxonomic keys, species synopses, flora and fauna studies, and data- this series is necessary in the transac- intensive reports on investigations in fishery science, engineering, and economics. tion of the public business required by law of this Department.