Loss of M1acp3ψ Ribosomal RNA Modification Is a Major Feature of Cancer
Total Page:16
File Type:pdf, Size:1020Kb
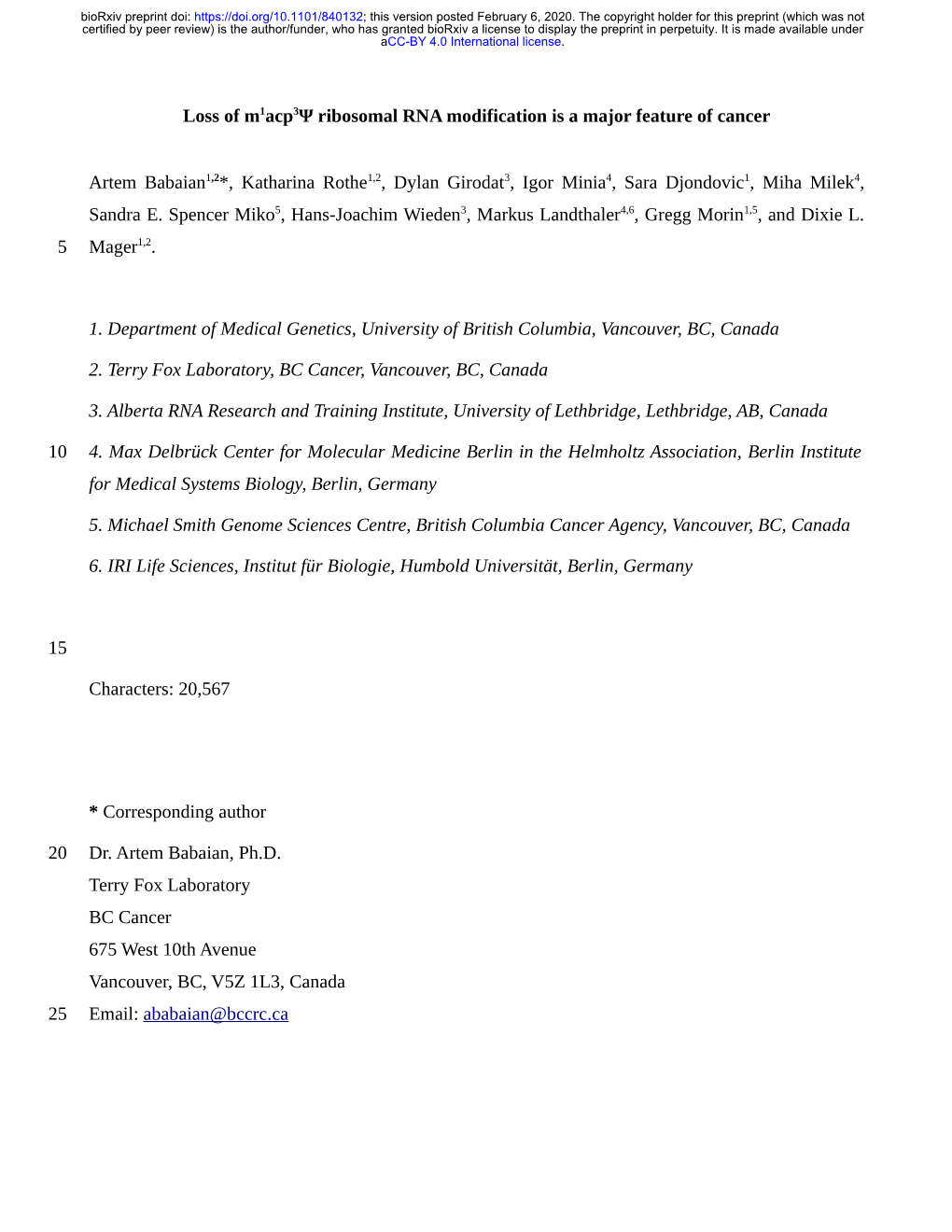
Load more
Recommended publications
-
Cryptococcus Neoformans Can Form Titan-Like Cells in Vitro in Response to Multiple Signals
RESEARCH ARTICLE Cryptococcus neoformans can form titan-like cells in vitro in response to multiple signals Nuria Trevijano-Contador1¤, Haroldo Cesar de Oliveira1,2, RocõÂo GarcõÂa-Rodas1, SueÂlen Andreia Rossi1, Irene Llorente1, A ngel Zaballos3, Guilhem Janbon4, JoaquõÂn Ariño5, O scar Zaragoza1* 1 Mycology Reference Laboratory, National Centre for Microbiology, Instituto de Salud Carlos III, Majadahonda, Madrid, Spain, 2 Universidade Estadual Paulista (UNESP), Faculdade de Ciências Farmacêuticas, CaÃmpus Araraquara, Departamento de AnaÂlises ClõÂnicas, LaboratoÂrio de Micologia ClõÂnica, Araraquara, São Paulo, Brazil, 3 Genomics Unit, Core Scientific Services, Instituto de Salud Carlos III, a1111111111 Majadahonda, Madrid, Spain, 4 Institut Pasteur, Unite Biologie des ARN des Pathogènes Fongiques, a1111111111 DeÂpartement de Mycologie, Paris, France, 5 Institut de Biotecnologia i Biomedicina and Departament de a1111111111 BioquõÂmica i Biologia Molecular, Universitat Autònoma de Barcelona, Cerdanyola del Vallès, Spain a1111111111 a1111111111 ¤ Current address: Albert Einstein College of Medicine, Bronx, New York, United States of America. * [email protected] Abstract OPEN ACCESS Cryptococcus neoformans is an encapsulated pathogenic yeast that can change the size of Citation: Trevijano-Contador N, de Oliveira HC, GarcõÂa-Rodas R, Rossi SA, Llorente I, Zaballos AÂ, the cells during infection. In particular, this process can occur by enlarging the size of the et al. (2018) Cryptococcus neoformans can form capsule without modifying the size of the cell body, or by increasing the diameter of the cell titan-like cells in vitro in response to multiple body, which is normally accompanied by an increase of the capsule too. This last process signals. PLoS Pathog 14(5): e1007007. https://doi. leads to the formation of cells of an abnormal enlarged size denominated titan cells. -
Molecular Genetic Characterization of Ptr Toxc-Tsc1 Interaction
MOLECULAR GENETIC CHARACTERIZATION OF PTR TOXC-TSC1 INTERACTION AND COMPARATIVE GENOMICS OF PYRENOPHORA TRITICI-REPENTIS A Dissertation Submitted to the Graduate Faculty of the North Dakota State University of Agriculture and Applied Science By Gayan Kanishka Kariyawasam In Partial Fulfillment of the Requirements for the Degree of DOCTOR OF PHILOSOPHY Major Department: Plant Pathology November 2018 Fargo, North Dakota North Dakota State University Graduate School Title MOLECULAR GENETIC CHARACTERIZATION OF PTR TOXC-TSC1 INTERACTION AND COMPARATIVE GENOMICS OF PYRENOPHORA TRITICI-REPENTIS By Gayan Kanishka Kariyawasam The Supervisory Committee certifies that this disquisition complies with North Dakota State University’s regulations and meets the accepted standards for the degree of DOCTOR OF PHILOSOPHY SUPERVISORY COMMITTEE: Dr. Zhaohui Liu Chair Dr. Shaobin Zhong Dr. Justin D. Faris Dr. Phillip E. McClean Dr. Timothy L. Friesen Approved: November 7, 2018 Jack Rasmussen Date Department Chair ABSTRACT Tan spot of wheat, caused by Pyrenophora tritici-repentis, is an economically important disease worldwide. The disease system is known to involve three pairs of interactions between fungal-produced necrotrophic effectors (NEs) and the wheat sensitivity genes, namely Ptr ToxA- Tsn1, Ptr ToxB-Tsc2 and Ptr ToxC-Tsc1, all of which result in susceptibility. Many lines of evidence also suggested the involvement of additional fungal virulence and host resistance factors. Due to the non-proteinaceous nature, Ptr ToxC, has not been purified and the fungal gene (s) controlling Ptr ToxC production is unknown. The objective for the first part of research is to map the fungal gene (s) controlling Ptr ToxC production. Therefore, A bi-parental fungal population segregating for Ptr ToxC production was first developed from genetically modified heterothallic strains of AR CrossB10 (Ptr ToxC producer) and 86-124 (Ptr ToxC non-producer), and then was genotyped and phenotyped. -
Glucosyltransferase B3GLCT Gene Mutated in Peters Plus Syndrome
RESEARCH ARTICLE Functional characterization of zebrafish orthologs of the human Beta 3- Glucosyltransferase B3GLCT gene mutated in Peters Plus Syndrome Eric Weh1,2¤a, Hideyuki Takeuchi3¤b, Sanaa Muheisen1, Robert S. Haltiwanger3, Elena V. Semina1,2,4* 1 Department of Pediatrics and Children's Research Institute at the Medical College of Wisconsin, a1111111111 Milwaukee, Wisconsin, United States of America, 2 Department of Cell Biology, Neurobiology, and Anatomy at the Medical College of Wisconsin, Milwaukee, Wisconsin, United States of America, 3 Complex a1111111111 Carbohydrate Research Center, University of Georgia, Athens, Georgia, United States of America, a1111111111 4 Department of Ophthalmology at the Medical College of Wisconsin, Milwaukee, Wisconsin, United States a1111111111 of America a1111111111 ¤a Current address: Department of Ophthalmology and Visual Sciences, W.K. Kellogg Eye Center, University of Michigan, Ann Arbor, MI, United States of America ¤b Current address: Department of Molecular Biochemistry, Nagoya University School of Medicine, Nagoya, Japan. * [email protected] OPEN ACCESS Citation: Weh E, Takeuchi H, Muheisen S, Haltiwanger RS, Semina EV (2017) Functional Abstract characterization of zebrafish orthologs of the human Beta 3-Glucosyltransferase B3GLCT gene Peters Plus Syndrome (PPS) is a rare autosomal recessive disease characterized by ocular mutated in Peters Plus Syndrome. PLoS ONE 12 defects, short stature, brachydactyly, characteristic facial features, developmental delay (9): e0184903. https://doi.org/10.1371/journal. pone.0184903 and other highly variable systemic defects. Classic PPS is caused by loss-of-function muta- tions in the B3GLCT gene encoding for a 3-glucosyltransferase that catalyzes the attach- Editor: Christoph Englert, Leibniz Institute on aging β - Fritz Lipmann Institute (FLI), GERMANY ment of glucose via a β1±3 glycosidic linkage to O-linked fucose on thrombospondin type 1 repeats (TSRs). -
Exploiting the Genetic Diversity of Wild Ancestors and Relatives of Wheat for Its Improvement Jagdeep Singh Sidhu South Dakota State University
South Dakota State University Open PRAIRIE: Open Public Research Access Institutional Repository and Information Exchange Electronic Theses and Dissertations 2018 Exploiting the Genetic Diversity of Wild Ancestors and Relatives of Wheat for its Improvement Jagdeep Singh Sidhu South Dakota State University Follow this and additional works at: https://openprairie.sdstate.edu/etd Part of the Plant Breeding and Genetics Commons Recommended Citation Sidhu, Jagdeep Singh, "Exploiting the Genetic Diversity of Wild Ancestors and Relatives of Wheat for its Improvement" (2018). Electronic Theses and Dissertations. 2641. https://openprairie.sdstate.edu/etd/2641 This Thesis - Open Access is brought to you for free and open access by Open PRAIRIE: Open Public Research Access Institutional Repository and Information Exchange. It has been accepted for inclusion in Electronic Theses and Dissertations by an authorized administrator of Open PRAIRIE: Open Public Research Access Institutional Repository and Information Exchange. For more information, please contact [email protected]. EXPLOITING THE GENETIC DIVERSITY OF WILD ANCESTORS AND RELATIVES OF WHEAT FOR ITS IMPROVEMENT BY JAGDEEP SINGH SIDHU A thesis submitted in partial fulfillment of the requirements for the Master of Science Major in Plant Science South Dakota State University 2018 iii This thesis is dedicated to my respected father Mr. Amrik Singh Sidhu, mother Mrs. Harjit Kaur, my dear sister Sukhdeep Kaur and cute niece Samreet. iv ACKNOWLEDGEMENTS First of all, I am grateful to Dr. Sunish Sehgal for giving me an opportunity work in his winter breeding program. My master’s work would not have been possible without his love, help, support and encouragement. I truly respect Dr. -
2019 Abstracts
We are delighted EDITORIAL to welcome the worldwide yeast community in Gothenburg for ICYGMB2019! The “International Yeast Conferences” started in the 1960s with a handful of delegates and since then have become THE most important event in yeast research. Now the yeast meeting to returns to Gothenburg. Many yeast researchers still remember the meeting in 2003 with over 1,100 delegates, a truly memorable event. The Life Sciences are changing, and yeast research remains at their forefront. Advancements in genome sequencing and genome editing just make yeast more exciting as model organism in basic cell biological research, genome evolution and as a tool for synthetic biology and biotechnology. One of the most important reasons for the enormous success of yeast research lies in the unique character of the international yeast research community. No other community employs such a free exchange and access to information and research tools. Nor has any other community had the ability to build – even intercontinental – consortia of critical mass to tackle large‐scale projects, such as in sequencing the first eukaryotic genome or the first comprehensive yeast knockout library. Yeast2019 is the meeting of the international yeast research community where the latest, and even unpublished results are exchanged, and new projects, alliances, and collaborations are founded. A do‐not‐miss‐event. We attempt to incorporate the present excitement in yeast research in the programme of yeast2019. We are confident that this conference will contain important news and information for all yeast researchers. Taken together, yeast2019 will provide an up‐ to‐date overview in yeast research and it will set the scene for years to come. -
Genome-Wide Association Mapping for Resistance to Leaf Rust, Stripe Rust
Theoretical and Applied Genetics https://doi.org/10.1007/s00122-018-3086-6 ORIGINAL ARTICLE Genome‑wide association mapping for resistance to leaf rust, stripe rust and tan spot in wheat reveals potential candidate genes Philomin Juliana1 · Ravi P. Singh2 · Pawan K. Singh2 · Jesse A. Poland3 · Gary C. Bergstrom4 · Julio Huerta‑Espino5 · Sridhar Bhavani6 · Jose Crossa2 · Mark E. Sorrells1 Received: 24 June 2017 / Accepted: 12 March 2018 © The Author(s) 2018 Abstract Key message Genome-wide association mapping in conjunction with population sequencing map and Ensembl plants was used to identify markers/candidate genes linked to leaf rust, stripe rust and tan spot resistance in wheat. Abstract Leaf rust (LR), stripe rust (YR) and tan spot (TS) are some of the important foliar diseases in wheat (Triticum aestivum L.). To identify candidate resistance genes for these diseases in CIMMYT’s (International Maize and Wheat Improvement Center) International bread wheat screening nurseries, we used genome-wide association studies (GWAS) in conjunction with information from the population sequencing map and Ensembl plants. Wheat entries were genotyped using genotyping-by-sequencing and phenotyped in replicated trials. Using a mixed linear model, we observed that seedling resistance to LR was associated with 12 markers on chromosomes 1DS, 2AS, 2BL, 3B, 4AL, 6AS and 6AL, and seedling resistance to TS was associated with 14 markers on chromosomes 1AS, 2AL, 2BL, 3AS, 3AL, 3B, 6AS and 6AL. Seed- ling and adult plant resistance (APR) to YR were associated with several markers at the distal end of chromosome 2AS. In addition, YR APR was also associated with markers on chromosomes 2DL, 3B and 7DS. -
ABSTRACT KALMAR, JACLYN. Development of Innovative
ABSTRACT KALMAR, JACLYN. Development of Innovative Strategies for the Analyses of Complex Biological Systems Using Mass Spectrometry. (Under the direction of Dr. David C. Muddiman). Mass spectrometry (MS) is a powerful analytical tool due to its versatility, specificity, and sensitivity. MS has allowed for the proliferation in collection of molecular level –omics data, which, in turn, has provided deep insights into various complex biological systems. This work discusses new strategies for the analysis of biological molecules thought be involved in the pathogenicity of Rice blast disease and Alzheimer’s disease. Infrared Matrix-Assisted Laser Desorption Electrospray Ionization (IR-MALDESI) mass spectrometry imaging was used to identify meta-metabolomic features of Magnaporthe oryzae infected barley leaves. Three separate sets of barley were inoculated with Wild type (WT) M. oryzae, an F-box E3 ligase protein knock out (E3 ligase KO) M. oryzae, or a control solution. Over the course of the infection, each treatment was imaged using an advanced polarity switching method, allowing the detection of low and high molecular weight compounds that ionize in positive or negative polarities. Serotonin, a barley defense metabolite, was putatively identified using MS1 data then confirmed with tandem mass spectrometry fragmentation patterns. Metabolites in the melanin pathway, important for infection development of M. oryzae, were also identified using MS1 data but were unable to be confirmed due to their low abundances. Molecules related to the pathogenicity of the fungus were only found in the samples treated with the wild type M. oryzae where those treated with the genetically modified version displayed no metabolic changes related to a fungal infection. -
Genomic and Transcriptomic Investigations Into the Feed Efficiency Phenotype of Beef Cattle
Provided by the author(s) and NUI Galway in accordance with publisher policies. Please cite the published version when available. Title Genomic and transcriptomic investigations into the feed efficiency phenotype of beef cattle Author(s) Higgins, Marc Publication Date 2019-03-06 Publisher NUI Galway Item record http://hdl.handle.net/10379/15008 Downloaded 2021-09-25T18:07:39Z Some rights reserved. For more information, please see the item record link above. Genomic and Transcriptomic Investigations into the Feed Efficiency Phenotype of Beef Cattle Marc Higgins, B.Sc., M.Sc. A thesis submitted for the Degree of Doctor of Philosophy to the Discipline of Biochemistry, School of Natural Sciences, National University of Ireland, Galway. Supervisor: Dr. Derek Morris Discipline of Biochemistry, School of Natural Sciences, National University of Ireland, Galway. Supervisor: Dr. Sinéad Waters Teagasc, Animal and Bioscience Research Department, Animal & Grassland Research and Innovation Centre, Teagasc, Grange. Submitted November 2018 Table of Contents Declaration ................................................................................................................ vii Funding .................................................................................................................... viii Acknowledgements .................................................................................................... ix Abstract ...................................................................................................................... -
WO 2016/040794 Al 17 March 2016 (17.03.2016) P O P C T
(12) INTERNATIONAL APPLICATION PUBLISHED UNDER THE PATENT COOPERATION TREATY (PCT) (19) World Intellectual Property Organization International Bureau (10) International Publication Number (43) International Publication Date WO 2016/040794 Al 17 March 2016 (17.03.2016) P O P C T (51) International Patent Classification: AO, AT, AU, AZ, BA, BB, BG, BH, BN, BR, BW, BY, C12N 1/19 (2006.01) C12Q 1/02 (2006.01) BZ, CA, CH, CL, CN, CO, CR, CU, CZ, DE, DK, DM, C12N 15/81 (2006.01) C07K 14/47 (2006.01) DO, DZ, EC, EE, EG, ES, FI, GB, GD, GE, GH, GM, GT, HN, HR, HU, ID, IL, IN, IR, IS, JP, KE, KG, KN, KP, KR, (21) International Application Number: KZ, LA, LC, LK, LR, LS, LU, LY, MA, MD, ME, MG, PCT/US20 15/049674 MK, MN, MW, MX, MY, MZ, NA, NG, NI, NO, NZ, OM, (22) International Filing Date: PA, PE, PG, PH, PL, PT, QA, RO, RS, RU, RW, SA, SC, 11 September 2015 ( 11.09.201 5) SD, SE, SG, SK, SL, SM, ST, SV, SY, TH, TJ, TM, TN, TR, TT, TZ, UA, UG, US, UZ, VC, VN, ZA, ZM, ZW. (25) Filing Language: English (84) Designated States (unless otherwise indicated, for every (26) Publication Language: English kind of regional protection available): ARIPO (BW, GH, (30) Priority Data: GM, KE, LR, LS, MW, MZ, NA, RW, SD, SL, ST, SZ, 62/050,045 12 September 2014 (12.09.2014) US TZ, UG, ZM, ZW), Eurasian (AM, AZ, BY, KG, KZ, RU, TJ, TM), European (AL, AT, BE, BG, CH, CY, CZ, DE, (71) Applicant: WHITEHEAD INSTITUTE FOR BIOMED¬ DK, EE, ES, FI, FR, GB, GR, HR, HU, IE, IS, IT, LT, LU, ICAL RESEARCH [US/US]; Nine Cambridge Center, LV, MC, MK, MT, NL, NO, PL, PT, RO, RS, SE, SI, SK, Cambridge, Massachusetts 02142-1479 (US). -
Supplementary Materials and Methods
Truncating mutations in YIF1B cause a progressive encephalopathy with various degrees of mixed movement disorder, microcephaly, and epilepsy. Item Type Article Authors AlMuhaizea, Mohammed; AlMass, Rawan; AlHargan, Aljouhra; AlBader, Anoud; Medico Salsench, Eva; Howaidi, Jude; Ihinger, Jacie; Karachunski, Peter; Begtrup, Amber; Segura Castell, Monica; Bauer, Peter; Bertoli-Avella, Aida; Kaya, Ibrahim H; AlSufayan, Jumanah; AlQuait, Laila; Chedrawi, Aziza; Arold, Stefan T.; Colak, Dilek; Barakat, Tahsin Stefan; Kaya, Namik Citation AlMuhaizea, M., AlMass, R., AlHargan, A., AlBader, A., Medico Salsench, E., Howaidi, J., … Kaya, N. (2020). Truncating mutations in YIF1B cause a progressive encephalopathy with various degrees of mixed movement disorder, microcephaly, and epilepsy. Acta Neuropathologica. doi:10.1007/ s00401-020-02128-8 Eprint version Post-print DOI 10.1007/s00401-020-02128-8 Publisher Springer Nature Journal Acta neuropathologica Rights Archived with thanks to Acta neuropathologica Download date 06/10/2021 17:47:21 Link to Item http://hdl.handle.net/10754/661480 SUPPLEMENTARY MATERIALS AND METHODS Human Subjects The study was approved by institutional review boards (RAC# 2120022) at King Faisal Specialist Hospital and Research Centre and written informed consents were taken from the participants. Nucleic Acid Extraction, PCR, and Sanger Sequencing Peripheral blood samples were used for DNA extraction using Gentra’s Puregene Blood Kit, and QIAamp Venlo, Netherlands. Nucleic acid quality and quantity were determined using NanoDrop ND-1000 (ThermoFisher Scientific, Inc., Waltham, MA, US) and 2100 Bioanalyzer (Agilent Technologies, Inc., Santa Clara, CA, US) equipment. Primers were designed using Primer 3 and tested on human control DNA. PCRs were performed according to standard protocols and successfully amplified PCR products were directly used for Sanger sequencing using one of the PCR primers tagged with M13 universal site. -
Biogenesis and Functions of Aminocarboxypropyluridine in Trna
ARTICLE https://doi.org/10.1038/s41467-019-13525-3 OPEN Biogenesis and functions of aminocarboxypropyluridine in tRNA Mayuko Takakura 1,2, Kensuke Ishiguro 1,2, Shinichiro Akichika 1, Kenjyo Miyauchi 1 & Tsutomu Suzuki 1* Transfer (t)RNAs contain a wide variety of post-transcriptional modifications, which play critical roles in tRNA stability and functions. 3-(3-amino-3-carboxypropyl)uridine (acp3U) is a 1234567890():,; highly conserved modification found in variable- and D-loops of tRNAs. Biogenesis and functions of acp3U have not been extensively investigated. Using a reverse-genetic approach supported by comparative genomics, we find here that the Escherichia coli yfiP gene, which we rename tapT (tRNA aminocarboxypropyltransferase), is responsible for acp3U formation in tRNA. Recombinant TapT synthesizes acp3U at position 47 of tRNAs in the presence of S- adenosylmethionine. Biochemical experiments reveal that acp3U47 confers thermal stability on tRNA. Curiously, the ΔtapT strain exhibits genome instability under continuous heat stress. We also find that the human homologs of tapT, DTWD1 and DTWD2, are responsible for acp3U formation at positions 20 and 20a of tRNAs, respectively. Double knockout cells of DTWD1 and DTWD2 exhibit growth retardation, indicating that acp3U is physiologically important in mammals. 1 Department of Chemistry and Biotechnology, Graduate School of Engineering, The University of Tokyo, 7-3-1 Hongo, Bunkyo-ku, Tokyo 113-8656, Japan. 2These authors contributed equally: Mayuko Takakura, Kensuke Ishiguro. *email: [email protected] NATURE COMMUNICATIONS | (2019) 10:5542 | https://doi.org/10.1038/s41467-019-13525-3 | www.nature.com/naturecommunications 1 ARTICLE NATURE COMMUNICATIONS | https://doi.org/10.1038/s41467-019-13525-3 he emerging field of epitranscriptomics has revealed the Reduction in the level of the acp modification causes accumula- chemical diversity and functional importance of RNA tion of 18S rRNA precursors and a reduction in the level of 40S T fi fi modi cations. -
Characterization of Erwinia Amylovora - Host Plant and - Biocontrol Agent Interactions
Zurich Open Repository and Archive University of Zurich Main Library Strickhofstrasse 39 CH-8057 Zurich www.zora.uzh.ch Year: 2013 Characterization of Erwinia amylovora - host plant and - biocontrol agent interactions Kamber, Tim Posted at the Zurich Open Repository and Archive, University of Zurich ZORA URL: https://doi.org/10.5167/uzh-89948 Dissertation Originally published at: Kamber, Tim. Characterization of Erwinia amylovora - host plant and - biocontrol agent interactions. 2013, University of Zurich, Faculty of Science. Characterization of Erwinia amylovora - Host Plant and - Biocontrol Agent Interactions Dissertation zur Erlangung der naturwissenschaftlichen Doktorwürde (Dr. sc. nat.) vorgelegt der Mathematisch-naturwissenschaftlichen Fakultät der Universität Zürich von Tim Kamber von Hauenstein-Ifenthal (SO) Promotionskomitee: Prof. Dr. Beat Keller (Vorsitz) Prof. Dr. Robert Dudler Dr. Brion Duffy (Leitung der Dissertation) Zürich, 2013 Table of contents Table of contents 3 Summary 6 Zusammenfassung 8 Chapter 1. General Introduction 11 Erwinia amylovora – causal agent of the fire blight disease 12 Pantoea vagans C9-1 – a biocontrol agent of E. amylovora 16 Malus x domestica – host plant of E. amylovora 17 Objectives 18 Chapter 2. Genomics and current genetic understanding of Erwinia amylovora and the fire blight antagonist Pantoea vagans 19 Abstract 20 Introduction 20 Erwinia amylovora genomics 21 Erwinia inter-species genomics 23 Selected features clarified using comparative genomics 28 Type III secretion systems 28 Exopolysaccharides