128 Identical Quantum Sources Integrated on a Single Silica Chip
Total Page:16
File Type:pdf, Size:1020Kb
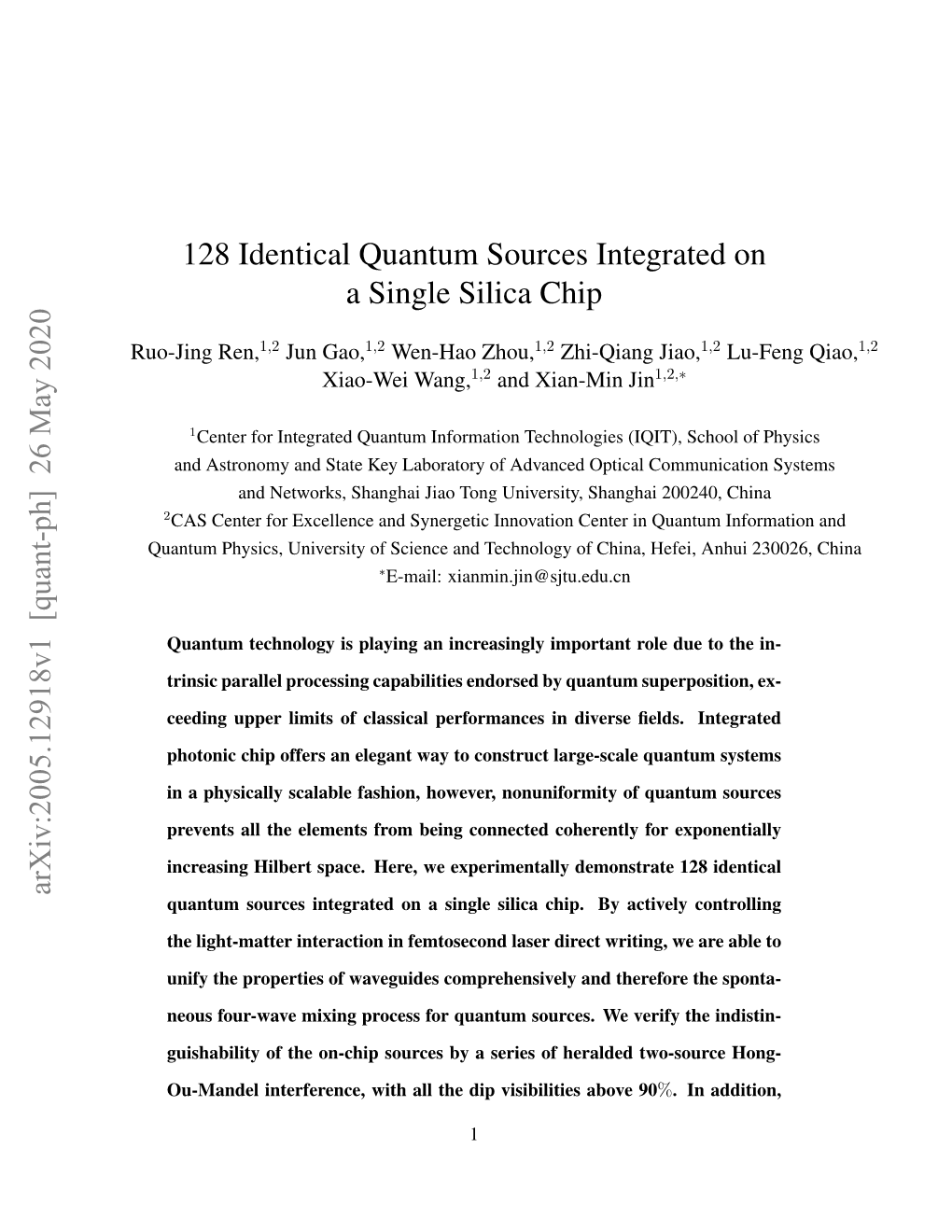
Load more
Recommended publications
-
A Radix-4 Chrestenson Gate for Optical Quantum Computation
A Radix-4 Chrestenson Gate for Optical Quantum Computation Kaitlin N. Smith, Tim P. LaFave Jr., Duncan L. MacFarlane, and Mitchell A. Thornton Quantum Informatics Research Group Southern Methodist University Dallas, TX, USA fknsmith, tlafave, dmacfarlane, mitchg @smu.edu Abstract—A recently developed four-port directional coupler is described in Section III followed by the realization of the used in optical signal processing applications is shown to be coupler with optical elements including its fabrication and equivalent to a radix-4 Chrestenson operator, or gate, in quantum characterization in Section IV. Demonstration of the four-port information processing (QIP) applications. The radix-4 qudit is implemented as a location-encoded photon incident on one of coupler as a radix-4 Chrestenson gate is presented in Section V the four ports of the coupler. The quantum informatics transfer and a summary with conclusions is found in Section VI. matrix is derived for the device based upon the conservation of energy equations when the coupler is employed in a classical II. QUANTUM THEORY BACKGROUND sense in an optical communications environment. The resulting transfer matrix is the radix-4 Chrestenson transform. This result A. The Qubit vs. Qudit indicates that a new practical device is available for use in the The quantum bit, or qubit, is the standard unit of information implementation of radix-4 QIP applications or in the construction for radix-2, or base-2, quantum computing. The qubit models of a radix-4 quantum computer. Index Terms—quantum information processing; quantum pho- information as a linear combination of two orthonormal basis tonics; qudit; states such as the states j0i and j1i. -
Electrical Characterisation of Ion Implantation Induced Defects in Silicon Based Devices for Quantum Applications
Electrical Characterisation of Ion Implantation Induced Defects in Silicon Based Devices for Quantum Applications Aochen Duan Supervised by Professor Jeffrey C. McCallum and Doctor Brett C. Johnson School of Physics The University of Melbourne Australia 1 Abstract Quantum devices that leverage the manufacturing techniques of silicon-based classical computers make them strong candidates for future quantum computers. However, the demands on device quality are much more stringent given that quantum states can de- cohere via interactions with their environment. In this thesis, a detailed investigation of ion implantation induced defects generated during device fabrication in a regime relevant to quantum device fabrication is presented. We identify different types of defects in Si using various advanced electrical characterisation techniques. The first experimental technique, electrical conductance, was used for the investigation of the interface state density of both n- and p-type MOS capacitors after ion implantation of various species followed by a rapid thermal anneal. As precise atomic placement is critical for building Si based quantum computers, implantation through the oxide in fully fabricated devices is necessary for some applications. However, implanting through the oxide might affect the quality of the Si/SiO2 interface which is in close proximity to the region in which manipulation of the qubits take place. Implanting ions in MOS capacitors through the oxide is a model for the damage that might be observed in other fabricated devices. It will be shown that the interface state density only changes significantly after a fluence of 1013 ions/cm2 except for Bi in p-type silicon, where significant increase in interface state density was observed after a fluence of 1011 Bi/cm2. -
Technology-Dependent Quantum Logic Synthesis and Compilation
Southern Methodist University SMU Scholar Electrical Engineering Theses and Dissertations Electrical Engineering Fall 12-21-2019 Technology-dependent Quantum Logic Synthesis and Compilation Kaitlin Smith Southern Methodist University, [email protected] Follow this and additional works at: https://scholar.smu.edu/engineering_electrical_etds Part of the Other Electrical and Computer Engineering Commons Recommended Citation Smith, Kaitlin, "Technology-dependent Quantum Logic Synthesis and Compilation" (2019). Electrical Engineering Theses and Dissertations. 30. https://scholar.smu.edu/engineering_electrical_etds/30 This Dissertation is brought to you for free and open access by the Electrical Engineering at SMU Scholar. It has been accepted for inclusion in Electrical Engineering Theses and Dissertations by an authorized administrator of SMU Scholar. For more information, please visit http://digitalrepository.smu.edu. TECHNOLOGY-DEPENDENT QUANTUM LOGIC SYNTHESIS AND COMPILATION Approved by: Dr. Mitchell Thornton - Committee Chairman Dr. Jennifer Dworak Dr. Gary Evans Dr. Duncan MacFarlane Dr. Theodore Manikas Dr. Ronald Rohrer TECHNOLOGY-DEPENDENT QUANTUM LOGIC SYNTHESIS AND COMPILATION A Dissertation Presented to the Graduate Faculty of the Lyle School of Engineering Southern Methodist University in Partial Fulfillment of the Requirements for the degree of Doctor of Philosophy with a Major in Electrical Engineering by Kaitlin N. Smith (B.S., EE, Southern Methodist University, 2014) (B.S., Mathematics, Southern Methodist University, 2014) (M.S., EE, Southern Methodist University, 2015) December 21, 2019 ACKNOWLEDGMENTS I am grateful for the many people in my life who made the completion of this dissertation possible. First, I would like to thank Dr. Mitch Thornton for introducing me to the field of quantum computation and for directing me during my graduate studies. -
Arxiv:Quant-Ph/0512071 V1 9 Dec 2005 Contents I.Ipoeet Ntekmprotocol KLM the on Improvements III
Linear optical quantum computing Pieter Kok,1,2, ∗ W.J. Munro,2 Kae Nemoto,3 T.C. Ralph,4 Jonathan P. Dowling,5,6 and G.J. Milburn4 1Department of Materials, Oxford University, Oxford OX1 3PH, UK 2Hewlett-Packard Laboratories, Filton Road Stoke Gifford, Bristol BS34 8QZ, UK 3National Institute of Informatics, 2-1-2 Hitotsubashi, Chiyoda-ku, Tokyo 101-8430, Japan 4Centre for Quantum Computer Technology, University of Queensland, St. Lucia, Queensland 4072, Australia 5Hearne Institute for Theoretical Physics, Dept. of Physics and Astronomy, LSU, Baton Rouge, Louisiana 6Institute for Quantum Studies, Department of Physics, Texas A&M University (Dated: December 9, 2005) Linear optics with photo-detection is a prominent candidate for practical quantum computing. The protocol by Knill, Laflamme and Milburn [Nature 409, 46 (2001)] explicitly demonstrates that efficient scalable quantum computing with single photons, linear optical elements, and projec- tive measurements is possible. Subsequently, several improvements on this protocol have started to bridge the gap between theoretical scalability and practical implementation. We review the original proposal and its improvements, and we give a few examples of experimental two-qubit gates. We discuss the use of realistic components, the errors they induce in the computation, and how they can be corrected. PACS numbers: 03.67.Hk, 03.65.Ta, 03.65.Ud Contents References 36 I. Quantum computing with light 1 A. Linear quantum optics 2 B. N-port interferometers and optical circuits 3 C. Qubits in linear optics 4 I. QUANTUM COMPUTING WITH LIGHT D. Early optical quantum computers and nonlinearities 5 Quantum computing has attracted much attention over II. -
Arxiv:1903.12615V1
Encoding an oscillator into many oscillators Kyungjoo Noh,1, 2, ∗ S. M. Girvin,1, 2 and Liang Jiang1, 2, y 1Departments of Applied Physics and Physics, Yale University, New Haven, Connecticut 06520, USA 2Yale Quantum Institute, Yale University, New Haven, Connecticut 06520, USA Gaussian errors such as excitation losses, thermal noise and additive Gaussian noise errors are key challenges in realizing large-scale fault-tolerant continuous-variable (CV) quantum information processing and therefore bosonic quantum error correction (QEC) is essential. In many bosonic QEC schemes proposed so far, a finite dimensional discrete-variable (DV) quantum system is encoded into noisy CV systems. In this case, the bosonic nature of the physical CV systems is lost at the error-corrected logical level. On the other hand, there are several proposals for encoding an infinite dimensional CV system into noisy CV systems. However, these CV-into-CV encoding schemes are in the class of Gaussian quantum error correction and therefore cannot correct practically relevant Gaussian errors due to established no-go theorems which state that Gaussian errors cannot be corrected by Gaussian QEC schemes. Here, we work around these no-go results and show that it is possible to correct Gaussian errors using GKP states as non-Gaussian resources. In particular, we propose a family of non-Gaussian quantum error-correcting codes, GKP-repetition codes, and demonstrate that they can correct additive Gaussian noise errors. In addition, we generalize our GKP-repetition codes to an even broader class of non-Gaussian QEC codes, namely, GKP-stabilizer codes and show that there exists a highly hardware-efficient GKP-stabilizer code, the two-mode GKP-squeezed-repetition code, that can quadratically suppress additive Gaussian noise errors in both the position and momentum quadratures. -
G53NSC and G54NSC Non Standard Computation Research Presentations
G53NSC and G54NSC Non Standard Computation Research Presentations March the 23rd and 30th, 2010 Tuesday the 23rd of March, 2010 11:00 - James Barratt • Quantum error correction 11:30 - Adam Christopher Dunkley and Domanic Nathan Curtis Smith- • Jones One-Way quantum computation and the Measurement calculus 12:00 - Jack Ewing and Dean Bowler • Physical realisations of quantum computers Tuesday the 30th of March, 2010 11:00 - Jiri Kremser and Ondrej Bozek Quantum cellular automaton • 11:30 - Andrew Paul Sharkey and Richard Stokes Entropy and Infor- • mation 12:00 - Daniel Nicholas Kiss Quantum cryptography • 1 QUANTUM ERROR CORRECTION JAMES BARRATT Abstract. Quantum error correction is currently considered to be an extremely impor- tant area of quantum computing as any physically realisable quantum computer will need to contend with the issues of decoherence and other quantum noise. A number of tech- niques have been developed that provide some protection against these problems, which will be discussed. 1. Introduction It has been realised that the quantum mechanical behaviour of matter at the atomic and subatomic scale may be used to speed up certain computations. This is mainly due to the fact that according to the laws of quantum mechanics particles can exist in a superposition of classical states. A single bit of information can be modelled in a number of ways by particles at this scale. This leads to the notion of a qubit (quantum bit), which is the quantum analogue of a classical bit, that can exist in the states 0, 1 or a superposition of the two. A number of quantum algorithms have been invented that provide considerable improvement on their best known classical counterparts, providing the impetus to build a quantum computer. -
High Fidelity Readout of Trapped Ion Qubits
High Fidelity Readout of Trapped Ion Qubits A thesis submitted for the degree of Doctor of Philosophy Alice Heather Burrell Trinity Term Exeter College 2010 Oxford ii Abstract High Fidelity Readout of Trapped Ion Qubits A thesis submitted for the degree of Doctor of Philosophy, Trinity Term 2010 Alice Heather Burrell Exeter College, Oxford This thesis describes experimental demonstrations of high-fidelity readout of trapped ion quantum bits (“qubits”) for quantum information processing. We present direct single-shot measurement of an “optical” qubit stored in a single 40Ca+ ion by the process of resonance fluorescence with a fidelity of 99.991(1)% (sur- passing the level necessary for fault-tolerant quantum computation). A time-resolved maximum likelihood method is used to discriminate efficiently between the two qubit states based on photon-counting information, even in the presence of qubit decay from one state to the other. It also screens out errors due to cosmic ray events in the detec- tor, a phenomenon investigated in this work. An adaptive method allows the 99.99% level to be reached in 145 µs average detection time. The readout fidelity is asymmetric: 99.9998% is possible for the “bright” qubit state, while retaining 99.98% for the “dark” state. This asymmetry could be exploited in quantum error correction (by encoding the “no-error” syndrome of the ancilla qubits in the “bright” state), as could the likelihood values computed (which quantify confidence in the measurement outcome). We then extend the work to parallel readout of a four-ion string using a CCD camera and achieve the same 99.99% net fidelity, limited by qubit decay in the 400 µs exposure time. -
Complexity Theory and Its Applications in Linear Quantum
Louisiana State University LSU Digital Commons LSU Doctoral Dissertations Graduate School 2016 Complexity Theory and its Applications in Linear Quantum Optics Jonathan Olson Louisiana State University and Agricultural and Mechanical College, [email protected] Follow this and additional works at: https://digitalcommons.lsu.edu/gradschool_dissertations Part of the Physical Sciences and Mathematics Commons Recommended Citation Olson, Jonathan, "Complexity Theory and its Applications in Linear Quantum Optics" (2016). LSU Doctoral Dissertations. 2302. https://digitalcommons.lsu.edu/gradschool_dissertations/2302 This Dissertation is brought to you for free and open access by the Graduate School at LSU Digital Commons. It has been accepted for inclusion in LSU Doctoral Dissertations by an authorized graduate school editor of LSU Digital Commons. For more information, please [email protected]. COMPLEXITY THEORY AND ITS APPLICATIONS IN LINEAR QUANTUM OPTICS A Dissertation Submitted to the Graduate Faculty of the Louisiana State University and Agricultural and Mechanical College in partial fulfillment of the requirements for the degree of Doctor of Philosophy in The Department of Physics and Astronomy by Jonathan P. Olson M.S., University of Idaho, 2012 August 2016 Acknowledgments My advisor, Jonathan Dowling, is apt to say, \those who take my take my advice do well, and those who don't do less well." I always took his advice (sometimes even against my own judgement) and I find myself doing well. He talked me out of a high-paying, boring career, and for that I owe him a debt I will never be able to adequately repay. My mentor, Mark Wilde, inspired me to work hard without saying a word about what I \should" be doing, and instead leading by example. -
Arxiv:Quant-Ph/0512071V2 14 Mar 2006 I Ulo:Byn Ieroptics Linear Beyond Outlook: VI
Linear optical quantum computing 1,2, 2 3 4 5, 6 4 Pieter Kok, ∗ W.J. Munro, Kae Nemoto, T.C. Ralph, Jonathan P. Dowling, and G.J. Milburn 1Department of Materials, Oxford University, Oxford OX1 3PH, UK 2Hewlett-Packard Laboratories, Filton Road Stoke Gifford, Bristol BS34 8QZ, UK 3National Institute of Informatics, 2-1-2 Hitotsubashi, Chiyoda-ku, Tokyo 101-8430, Japan 4Centre for Quantum Computer Technology, University of Queensland, St. Lucia, Queensland 4072, Australia 5Hearne Institute for Theoretical Physics, Department of Physics and Astronomy, LSU, Baton Rouge LA, 70803, USA 6Institute for Quantum Studies, Department of Physics, Texas A&M University, 77843-4242, USA (Dated: February 1, 2008) Linear optics with photon counting is a prominent candidate for practical quantum computing. The protocol by Knill, Laflamme, and Milburn [Nature 409, 46 (2001)] explicitly demonstrates that efficient scalable quantum computing with single photons, linear optical elements, and projective measure- ments is possible. Subsequently, several improvements on this protocol have started to bridge the gap between theoretical scalability and practical implementation. We review the original theory and its improvements, and we give a few examples of experimental two-qubit gates. We discuss the use of realistic components, the errors they induce in the computation, and how these errors can be corrected. PACS numbers: 03.67.Hk, 03.65.Ta, 03.65.Ud Contents I. QUANTUM COMPUTING WITH LIGHT I. Quantum computing with light 1 Quantum computing has attracted much attention over A. Linear quantum optics 2 B. N port interferometers and optical circuits 4 the last ten to fifteen years, partly because of its promise C. -
Arxiv:1002.4788V1 [Quant-Ph]
Laser & Photonics Reviews, August 29, 2018 1 Abstract This article reviews recent hybrid approaches to op- tical quantum information processing, in which both discrete and continuous degrees of freedom are exploited. There are well-known limitations to optical single-photon-based qubit and multi-photon-based qumode implementations of quantum communication and quantum computation, when the toolbox is restricted to the most practical set of linear operations and resources such as linear optics and Gaussian operations and states. The recent hybrid approaches aim at pushing the fea- sibility, the efficiencies, and the fidelities of the linear schemes Schematics for the optical implementation of entanglement dis- to the limits, potentially adding weak or measurement-induced nonlinearities to the toolbox. tribution between two stations in a hybrid quantum repeater. Copyright line will be provided by the publisher Optical hybrid approaches to quantum information Peter van Loock Optical Quantum Information Theory Group, Max Planck Institute for the Science of Light, Institute of Theoretical Physics I, Universit¨at Erlangen-N¨urnberg, Staudtstr.7/B2, 91058 Erlangen, Germany Received: . Published online: . Key words: quantum computation, quantum communication, quantum optics, entanglement, qubits, qumodes, hybrid Contents tion and quantum communication. More precisely, multi- partite entangled, so-called cluster states are a sufficient 1. Introduction ...................... 1 resource for universal, measurement-based quantum com- 2. Optical quantum information . 3 putation [1]. Further, the sequential distribution of many 3. Hybrid approaches . 13 copies of entangled states in a quantum repeater allow for 4. Summary and outlook . 31 extending quantum communicationto large distances, even when the physical quantum channel is imperfect such as a lossy, optical fiber [2,3]. -
Optical Quantum Computation: Information Processing Using Discrete- and Continuous-Variable States of Light
Optical Quantum Computation: Information Processing using Discrete- and Continuous-Variable States of Light By Luis An´ıbal Garc´ıaL´opez B.Sc., Universidad de los Andes, 2007 Advisor Prof. Jagdish R. Luthra, Ph.D. THESIS Submitted in Partial Fulfillment of the Requirements for the Degree of Master of Science Physics Universidad de los Andes, Departamento de F´ısica Bogot´a, Colombia December 7, 2009 Abstract Optical quantum computing uses measurement-based quantum computing schemes, with photons as physical qubits in the discrete case; it also takes advantage of the storage power of information available in the quadratures of the electromagnetic field that allows computation in a continuous-variable setting of qunats, or units of analogic information. For the first case, the main physical architecture for computation is based on a proposal by Knill, Laflamme and Milburn, the KLM architecture. In the continuous case, the process of encoding changes substantially, as we are not working with qubits anymore; the process of computation changes respectively by redefining the set of gates as a set of operations over the continuous states of the optical quadratures. We will present an overview of the field of optical quantum computing both in the discrete case, focusing in the KLM protocol of computation, and in the continuous setting, which enables the implementation of some quantum algorithms in a more natural way by means of the continuous quantum Fourier transform. Exploiting the advantages of both schemes, we shall describe a form of hybrid computation that involves optical qubits and qunats in the processing of information and apply it in the construction of the Deutsch-Jozsa algorithm. -
Fundamental Limits on Key Rates in Device-Independent Quantum Key Distribution
Fundamental limits on key rates in device-independent quantum key distribution Eneet Kaur∗ Mark M. Wilde∗ Andreas Wintery March 2, 2020 Abstract In this paper, we introduce intrinsic non-locality and quantum intrinsic non-locality as quan- tifiers for Bell non-locality, and we prove that they satisfy certain desirable properties such as faithfulness, convexity, and monotonicity under local operations and shared randomness. We then prove that intrinsic non-locality is an upper bound on the secret-key-agreement capacity of any device-independent protocol conducted using a device characterized by a correlation p, while quantum intrinsic non-locality is an upper bound on the same capacity for a correlation arising from an underlying quantum model. We also prove that intrinsic steerability is faithful, and it is an upper bound on the secret-key-agreement capacity of any one-sided device-independent protocol conducted using a device characterized by an assemblageρ ^. Finally, we prove that quantum intrinsic non-locality is bounded from above by intrinsic steerability. 1 Introduction In principle, quantum key distribution (QKD) [BB84, Eke91, SBPC+09] provides unconditional security [May01, SP00, LCT14] for establishing secret key at a distance. In the standard QKD setting, Alice and Bob (two spatially separated parties) trust the functioning of their devices. That is, it is assumed that they know the ensemble of states that their sources are preparing and the measurement that their devices are performing. However, this is a very strong set of assumptions. It is possible to consider other scenarios in which the trust assumptions are relaxed while still obtaining unconditional security.