A Theoretical Study of the Effect of Molecular Absorption and Re
Total Page:16
File Type:pdf, Size:1020Kb
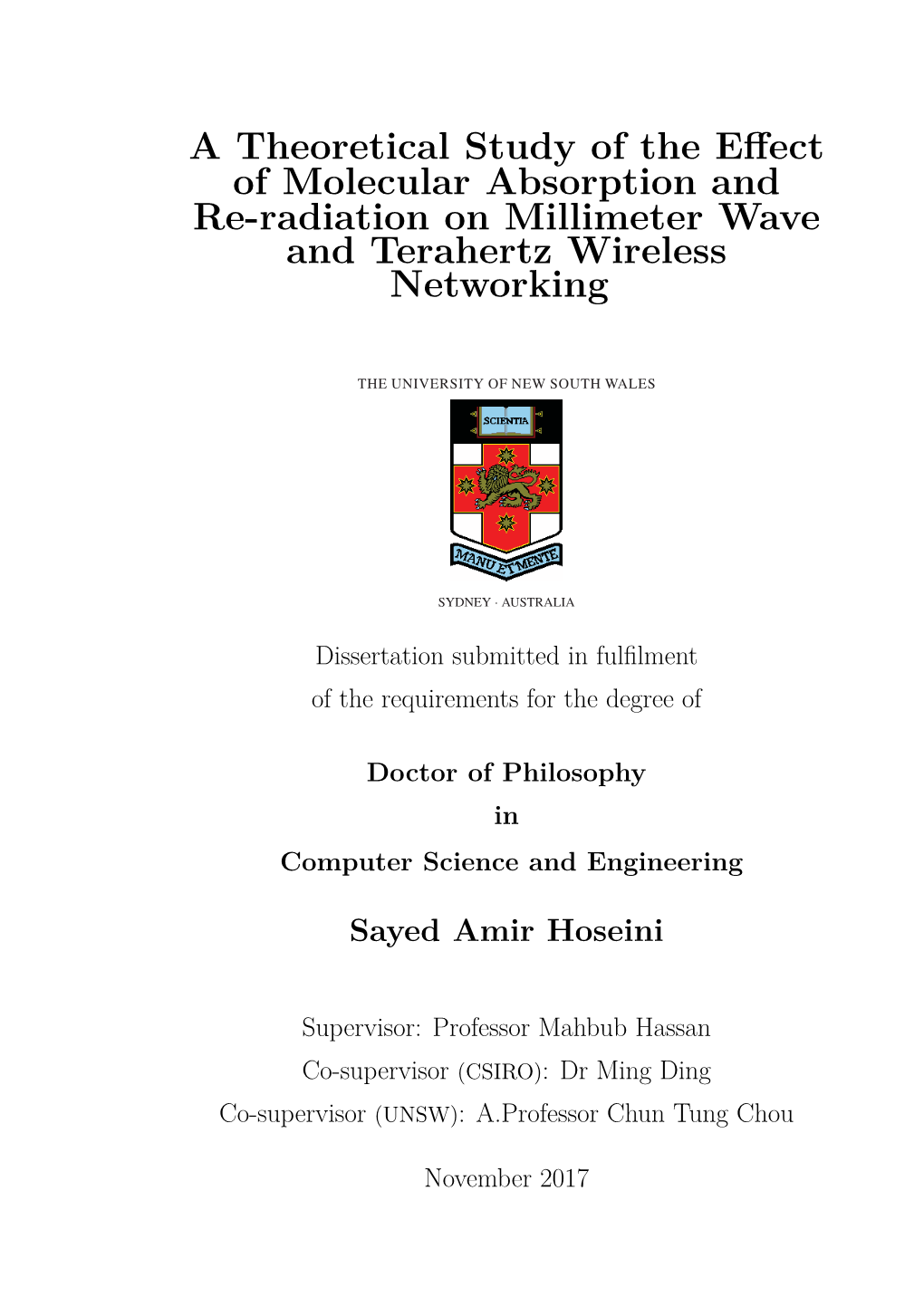
Load more
Recommended publications
-
RTT TECHNOLOGY TOPIC January 2015 Defence Spectrum – the New Battleground?
RTT TECHNOLOGY TOPIC January 2015 Defence Spectrum – the new battleground? In this month’s technology topic we look at contemporary military radio developments, the integration of LTE user devices into defence communication systems, the relevance of military research to 5 G deployment efficiency and related spectral utilisation and regulatory issues. Defence communication systems are deployed across the whole radio spectrum from long wave to light. This includes mobile communication systems at VHF and UHF and L Band and S band, LEO, MEO and GSO satellite systems (VHF to E band) and mobile and fixed radar (VHF to E band). Legacy defence systems are being upgraded to provide additional functionality. This requires more rather than less spectrum. Increased radar resolution requires wider channel bandwidths; longer range requires more power and improved sensitivity. Improved sensitivity increases the risk of inter system interference. Emerging application requirements including unmanned aerial vehicles require a mix of additional terrestrial, satellite and radar bandwidth. These requirements are geographically and spectrally diverse rather than battlefield and spectrally specific. The assumption in many markets is that the defence industry will be willing and able to surrender spectrum for mobile broadband consumer and civilian use. The AWS 3 auction in the US is a contemporary example with a $5 billion transition budget to cover legacy military system decommissioning in the DOD coordination zone between 1755 and 1780 MHz This transition strategy assumes an increased use of LTE network hardware and user hardware in battlefield systems. While this might imply an opportunity for closer coordination and cooperation between the mobile broadband and defence community it seems likely that an increase in the amount of defence bandwidth needed to support a broadening range of RF dependent systems could be a problematic component in the global spectral allocation and auction process. -
Wireless Backhaul Evolution Delivering Next-Generation Connectivity
Wireless Backhaul Evolution Delivering next-generation connectivity February 2021 Copyright © 2021 GSMA The GSMA represents the interests of mobile operators ABI Research provides strategic guidance to visionaries, worldwide, uniting more than 750 operators and nearly delivering actionable intelligence on the transformative 400 companies in the broader mobile ecosystem, including technologies that are dramatically reshaping industries, handset and device makers, software companies, equipment economies, and workforces across the world. ABI Research’s providers and internet companies, as well as organisations global team of analysts publish groundbreaking studies often in adjacent industry sectors. The GSMA also produces the years ahead of other technology advisory firms, empowering our industry-leading MWC events held annually in Barcelona, Los clients to stay ahead of their markets and their competitors. Angeles and Shanghai, as well as the Mobile 360 Series of For more information about ABI Research’s services, regional conferences. contact us at +1.516.624.2500 in the Americas, For more information, please visit the GSMA corporate +44.203.326.0140 in Europe, +65.6592.0290 in Asia-Pacific or website at www.gsma.com. visit www.abiresearch.com. Follow the GSMA on Twitter: @GSMA. Published February 2021 WIRELESS BACKHAUL EVOLUTION TABLE OF CONTENTS 1. EXECUTIVE SUMMARY ................................................................................................................................................................................5 -
ATHENA NGSO SATELLITE EXHIBIT 1 Technical Information To
REDACTED FOR PUBLIC INSPECTION ATHENA NGSO SATELLITE EXHIBIT 1 Technical Information to Supplement Form 442 and Application Narrative A.1 Scope and Purpose This exhibit supplements FCC Form 442 and contains the technical information referenced in the application narrative that is required by Parts 5 and 25 of the Commission’s rules. A.2 Radio Frequency Plan (§25.114(c)(4)) The Athena satellite will have two E-band uplinks and two E-band downlinks. The downlink emissions are nominally centered at 72 GHz and 75 GHz and the uplink emissions are nominally centered at 82 GHz and 85 GHz1. The bandwidth for both the uplinks and downlinks is 2.1852 GHz. The TT&C uplink will be conducted at 2082 MHz with an occupied bandwidth of 1.5 MHz. The TT&C downlink will be conducted at 8496.25 MHz with an occupied bandwidth of 2.3 MHz. Table A.2-1 shows the frequency ranges to be used by the Athena satellite. 1 There is the possibility that mild tuning may be performed from the planned 72, 75, 82 and 85 GHz centered carriers (e.g., 74.8 and 82.2 GHz may be used for example to mitigate any potential, mild “inter-channel interference” due to spectral regrowth issues and limited transmit- to-receive isolation). In addition, a limited number of tests, estimated at one to two dozen, may be performed with continuous wave (CW), unmodulated carriers as far out as the band edges (i.e., 71-76 GHz and 81-82 GHz) to measure the atmospheric attenuation characteristics. -
Terahertz Band: the Last Piece of RF Spectrum Puzzle for Communication Systems Hadeel Elayan, Osama Amin, Basem Shihada, Raed M
1 Terahertz Band: The Last Piece of RF Spectrum Puzzle for Communication Systems Hadeel Elayan, Osama Amin, Basem Shihada, Raed M. Shubair, and Mohamed-Slim Alouini Abstract—Ultra-high bandwidth, negligible latency and seamless communication for devices and applications are envisioned as major milestones that will revolutionize the way by which societies create, distribute and consume information. The remarkable expansion of wireless data traffic that we are witnessing recently has advocated the investigation of suitable regimes in the radio spectrum to satisfy users’ escalating requirements and allow the development and exploitation of both massive capacity and massive connectivity of heterogeneous infrastructures. To this end, the Terahertz (THz) frequency band (0.1-10 THz) has received noticeable attention in the research community as an ideal choice for scenarios involving high-speed transmission. Particularly, with the evolution of technologies and devices, advancements in THz communication is bridging the gap between the millimeter wave (mmW) and optical frequency ranges. Moreover, the IEEE 802.15 suite of standards has been issued to shape regulatory frameworks that will enable innovation and provide a complete solution that crosses between wired and wireless boundaries at 100 Gbps. Nonetheless, despite the expediting progress witnessed in THz Fig. 1. Wireless Roadmap Outlook up to the year 2035. wireless research, the THz band is still considered one of the least probed frequency bands. As such, in this work, we present an up-to-date review paper to analyze the fundamental elements I. INTRODUCTION and mechanisms associated with the THz system architecture. THz generation methods are first addressed by highlighting The race towards improving human life via developing the recent progress in the electronics, photonics as well as different technologies is witnessing a rapid pace in diverse plasmonics technology. -
Qatar National Frequency Allocation Plan and Specific
Communications Regulatory Authority 2 Table of Contents Qatar National Frequency Allocation Plan and Specific Assignments Table of Contents Part 01. GENERAL INFORMATION .............................................................................................................. 1. Introduction ...................................................................................................................................................5 2. Principals of Spectrum Management .................................................................................................5 3. Definition of terms used ..........................................................................................................................7 4. How to read the frequency allocation table .................................................................................. 11 5. Radio Wave Spectrum ............................................................................................................................ 12 Part 02. FREQUENCY ALLOCATION PLAN ............................................................................................... Qatar Frequency Allocation Plan ............................................................................................................ 15 Part 03. QATAR’S FOOTNOTES ................................................................................................................... Footnotes Relevant to Qatar................................................................................................................. -
The Need for E-Band
Sky Light Research Mobile World Congress 2013 Mobile Data Backhaul: The Need For E‐Band by Emmy Johnson We’ve been hearing about the bandwidth bottleneck for years, and as LTE and more broadband intensive applications are rolled out, rapidly increasing mobile data traffic will continue to threaten the stability of the network. This is especially a critical issue in urban centers where subscribers and mobile data traffic are the most concentrated. Thanks to the increasing popularity of smart phones from the likes of Apple and Samsung, mobile data is expected to continue to grow unabated the foreseeable future. Cisco’s most recent mobile data forecast predicted that mobile data will grow 13x over the next five years, from 0.9 Exabytes per month in 2012 to 11.2 Exabytes in 2017. Thus the question becomes, how do operators keep up with this level of exponential growth while keeping mobile services on par with customers’ expectations? There are several ways that operators are combatting this growth, through innovation in both the RAN and backhaul segments of the networks. The concept of small cells through heterogeneous networks is undoubtedly the most touted modernization, and although this is new, and very promising, there are still regulatory and cost details that need to be worked out to create cost-effective ROIs, especially in the outdoor model. In the interim, another, but not as sexy, solution exists in the backhaul - replacing legacy SDH microwave links with faster, packet based millimeterwave radio links. While it’s true that fiber is the preferred mode of backhauling traffic, it is not always available and cost effective. -
UV-Vis) Absorption Vs
授課教師: Professor 吳逸謨 教授 Warning: Copyrighted by textbook publisher. Do not use outside class. Principles of Instrumental Analysis Section III – Molecular Spectroscopy Chapter 13 Introduction to Ultraviolet-Visible Absorption Spectrometry + Chapter 14 Applications of Ultraviolet-Visible Absorption Spectrometry Reference: p. 423, Visible and UV spectra, in “Organic Chemistry” textbook – Solomons, 3rd Ed] 1 Molecular Spectroscopy Ultraviolet–visible (UV-Vis) absorption vs. fluorescence Spectroscopy Ultraviolet–visible spectroscopy or ultraviolet-visible spectrophotometry (UV-Vis or UV/Vis) refers to absorption spectroscopy or reflectance spectroscopy in the ultraviolet-visible spectral region. This means it uses light in the visible and adjacent (near-UV and near- infrared [NIR]) ranges. The absorption or reflectance in the visible range directly affects the perceived color of the chemicals involved. In this region of the electromagnetic spectrum, molecules undergo electronic transitions. Fluorescence spectroscopy is based on molecular emission: The “UV-Vis” technique is complementary to fluorescence spectroscopy, in that fluorescence deals with transitions from the excited state to the ground state, while UV-Vis absorption measures transitions from the ground state to the excited state.[1] 2 (2007/3) UV-Vis Spec 儀器 -實驗課 3 FIGURE 6-3 Regions of the electromagnetic spectrum. (For UV-Vis, λ = 100~500 nm, 500~1000 nm) 4 Ch6 An Introduction to Spectrometric Methods P.135 Apendix: Chap. 7E - Radiation Transducers p. 191 Read the texts in Chap. 7 7E-2 Photon Transducers for optical spectroscopy – Barrier-Layer Photovoltaic Cells – Vacuum Phototubes – Photomultiplier tubes (PMT), picture on p. 195 – Silicon Photodiodes (Fig. 7-32) [semiconductor type] [A silicon photodiode transducer consists of a reverse- biased p-n junction.] (The latter two types of transducers are more commonly used in UV-Vis.) – We will discuss more details later. -
A Photonically-Excited Leaky-Wave Antenna Array at E-Band for 1-D Beam Steering
applied sciences Article A Photonically-Excited Leaky-Wave Antenna Array at E-Band for 1-D Beam Steering Álvaro J. Pascual-Gracia 1, Muhsin Ali 2 , Guillermo Carpintero Del Barrio 2 , Fabien Ferrero 3, Laurent Brochier 3, Ronan Sauleau 1, Luis Enrique García-Muñoz 2,* and David González-Ovejero 1,* 1 Univ Rennes, CNRS, IETR (Institut d’Electronique et de Télécommunications de Rennes)–UMR 6164, F-35000 Rennes, France; [email protected] (Á.J.P.-G.); [email protected] (R.S.) 2 Universidad Carlos III de Madrid, 28911 Leganés, Spain; [email protected] (M.A.); [email protected] (G.C.D.B.) 3 Université Côte d’Azur, CNRS, LEAT (Laboratory of Electronics Antennas and Telecommunications)–UMR 7248, 06903 Sophia Antipolis, France; [email protected] (F.F.); [email protected] (L.B.) * Correspondence: [email protected] (L.E.G.-M.); [email protected] (D.G.-O.) Received: 30 March 2020; Accepted: 14 May 2020; Published: 18 May 2020 Abstract: This manuscript reports the first leaky-wave antenna (LWA) array excited by a photomixer as well as its potential application for alignment in wireless links. The designed array is manufactured in printed circuit board (PCB) technology, works at the E-band (from 75 to 85 GHz), and provides a directive beam of about 18 dBi with a frequency scanning span of 22◦. The antenna element consists of a microstrip line periodically loaded with stubs, and it has been designed employing a hybrid approach combining full-wave simulations and transmission line theory. -
A Review of Advanced CMOS RF Power Amplifier Architecture Trends
electronics Review A Review of Advanced CMOS RF Power Amplifier Architecture Trends for Low Power 5G Wireless Networks Aleksandr Vasjanov 1,2,* and Vaidotas Barzdenas 1,2 1 Department of Computer Science and Communications Technologies, Vilnius Gediminas Technical University, 10221 Vilnius, Lithuania; [email protected] 2 Micro and Nanoelectronics Systems Design and Research Laboratory, Vilnius Gediminas Technical University, 10257 Vilnius, Lithuania * Correspondence: [email protected]; Tel.: +370-5-274-4769 Received: 15 September 2018; Accepted: 19 October 2018; Published: 23 October 2018 Abstract: The structure of the modern wireless network evolves rapidly and maturing 4G networks pave the way to next generation 5G communication. A tendency of shifting from traditional high-power tower-mounted base stations towards heterogeneous elements can be spotted, which is mainly caused by the increase of annual wireless users and devices connected to the network. The radio frequency (RF) power amplifier (PA) performance directly affects the efficiency of any transmitter, therefore, the emerging 5G cellular network requires new PA architectures with improved efficiency without sacrificing linearity. A review of the most promising reported RF PA architectures is presented in this article, emphasizing advantages, disadvantages and concluding with a quantitative comparison. The main scope of reviewed papers are PAs implemented in scalable complementary metal–oxide–semiconductor (CMOS) and SiGe BiCMOS processes with output powers suitable for portable wireless devices under 32 dBm (1.5 W) in the low- and high- 5G network frequency ranges. Keywords: power amplifier; architecture; radio frequency; wireless; network; 5G; trends 1. Introduction The first most primitive radio transmitter that was used for telegraphy was developed in the early 1890s by Guglielmo Marconi. -
Towards Sensitive Terahertz Detection Via Thermoelectric Manipulation
Liu et al. NPG Asia Materials (2018) 10: 318–327 DOI 10.1038/s41427-018-0032-7 NPG Asia Materials ARTICLE Open Access Towards sensitive terahertz detection via thermoelectric manipulation using graphene transistors Changlong Liu1,2,LeiDu1,2,WeiweiTang1,2,DachengWei3,JinhuaLi4,LinWang1,2,5,GangChen1,2, Xiaoshuang Chen1,2,5 and Wei Lu1,2,5 Abstract Graphene has been highly sought after as a potential candidate for hot-electron terahertz (THz) detection benefiting from its strong photon absorption, fast carrier relaxation, and weak electron-phonon coupling. Nevertheless, to date, graphene-based thermoelectric THz photodetection is hindered by low responsivity owing to relatively low photoelectric efficiency. In this work, we provide a straightforward strategy for enhanced THz detection based on antenna-coupled CVD graphene transistors with the introduction of symmetric paired fingers. This design enables switchable photodetection modes by controlling the interaction between the THz field and free hot carriers in the graphene-channel through different contacting configurations. Hence a novel “bias-field effect” can be activated, which leads to a drastic enhancement in THz detection ability with maximum responsivity of up to 280 V/W at 0.12 THz relative to the antenna area and a Johnson-noise limited minimum noise-equivalent power (NEP) of 100 pW/Hz0.5 at room temperature. The mechanism responsible for the enhancement in the photoelectric gain is attributed to thermophotovoltaic instead of plasma self-mixing effects. Our results offer a promising alternative route toward 1234567890():,; 1234567890():,; scalable, wafer-level production of high-performance graphene detectors. Introduction interact with light10,11, which indicates they are promising Recently, two-dimensional (2D) materials (transition materials with great potential in optoelectronic devices for metal dichalcogenides (TMDs), graphene, black phos- communication, sensing, and imaging, particularly in the phorus, etc.) have ignited intensive interest due to their visible and near-infrared range. -
Notice of Proposed Rulemaking and Order, WT Docket No
May 19, 2020 FCC FACT SHEET* Modernizing and Expanding Access to the 70/80/90 GHz Bands Notice of Proposed Rulemaking and Order, WT Docket No. 20-133, et al. Background: This Notice of Proposed Rulemaking initiates a proceeding to explore innovative new commercial uses of the 71–76 GHz, 81–86 GHz, 92–94 GHz, and 94.1–95 GHz bands (collectively, the “70/80/90 GHz bands”), which are allocated to co-primary non-Federal and Federal use. 70/80/90 GHz band licensees currently use this spectrum for fixed, point-to-point communications links but the spectrum is unused (or minimally used) in large parts of the United States. This underuse, combined with recent technological developments, makes the 70/80/90 GHz bands a potential resource for several categories of new and innovative service offerings, especially wireless 5G backhaul and broadband services on-board aircraft and ships, in furtherance of the Commission’s 5G FAST Plan. What the Notice of Proposed Rulemaking Would Do: • Propose changes to antenna standards for the 70 and 80 GHz bands to permit the use of smaller antennas and seek comment on whether to make similar changes in the 90 GHz band. • Propose to authorize point-to-point links to endpoints in motion in the 70 and 80 GHz bands and classify those links as “mobile” service. • Seek comment on whether the Commission should change its link registration rules for the 70/80/90 GHz bands to eliminate never-constructed links from third-party registration databases. • Seek comment on any technical and operational rules necessary to allow new service offerings in the 70 and 80 GHz bands and to mitigate interference to both incumbents and other proposed users of these bands. -
UV Spectroscopy
Electronic Excitation by UV/Vis Spectroscopy : UV: IR: Radio waves: X-ray: valance core molecular Nuclear spin states electron electronic vibrations (in a magnetic field) excitation excitation • Interaction of electromagnetic radiant with matter – The wave-length, l, and the wave number, v’, of e.m.r. changes with the medium it travels through, because of the refractive index of the medium; the frequency, v, however, remains unchanged – Types of interactions •Absorption absorption •Reflection transmissio n •Transmission reflectio scatterin refractio •Scattering n g n •Refraction – Each interaction can disclose certain properties of the matter – When applying e.m.r. of different frequency (thus the energy e.m.r. carried) different type information can be obtained Spectral properties, applications, and interactions of electromagnetic radiation Wave number Type of Energy Wavelength Frequency Type of Type of v’ l v quantum Electron -1 cm radiation spectroscopy kcal/mol vole eV cm Hz transitio n Gamma 9.4x107 4.1x106 3.3x1010 3.0x10-11 1021 Gamma ray ray emission Nuclear X-ray 5 4 8 -9 19 Electronic 9.4x10 4.1x10 3.3x10 3.0x10 10 absorption (inner shell) X-ray emission 9.4x103 4.1x102 3.3x106 3.0x10-7 1017 Ultra Violet Vac UV absorption absorption 1 0 4 -5 15 UV emission Electronic 9.4x10 4.1x10 3.3x10 3.0x10 10 Visible Vis fluorescence (outer shell) Infrared IR absorption -1 -2 2 -3 13 Raman 9.4x10 4.1x10 3.3x10 3.0x10 10 Molecular vibration -3 -4 0 -1 11 Molecular 9.4x10 4.1x10 3.3x10 3.0x10 10 Microwave Microwave absorption rotation 9.4x10-5 4.1x10-6 3.3x10-2 3.0x101 109 Electron paramagnet Magnetically resonance induced spin 9.4x10-7 4.1x10-8 3.3x10-4 3.0x103 107 Nuclear magnetic states Radio resonance UV Spectroscopy I.